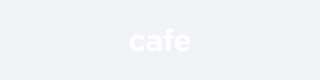
The eccrine gland is one of the major cutaneous appendages and secretes sweat. Its principal function is thermoregulation during exposure to a hot environment or physical exercise. In addition to this function, we show that LL-37, a member of cathelicidin family of anti-microbial peptides, is expressed in sweat. LL-37 protein and mRNA was seen in the eccrine structures of normal human skin by immunohistochemistry and in situ hybridization. LL-37 was localized to both the eccrine gland and sweat ductal epithelial cells, whereas dermcidin, a previously described natural antibiotic in sweat, was expressed only in the gland itself. The anti-microbial activity of LL-37 and dermcidin against various bacteria in the sweat ionic environment was demonstrated by solution colony forming assay using synthetic peptides, and in sweat obtained from normal volunteers. These results indicate that cathelicidin is secreted in human sweat, has potent anti-microbial activity against both gram-positive and gram-negative bacteria, and can, after processing from the preproform, provide a barrier for protection against infection. Thus, sweat represents a unique mode of delivery for potent innate immune effector molecules in the absence of inflammation.
에크린선은 주요 피부 부속기관 중 하나이며 땀을 분비합니다. 에크린선의 주요 기능은 더운 환경에 노출되거나 운동을 하는 동안 체온을 조절하는 것입니다. 이 기능 외에도 항균 펩타이드의 일종인 카탈리시딘 계열의 LL-37이 땀에서 발현된다는 것을 보여주었습니다. 면역조직화학 및 현장 혼성화를 통해 정상인 피부의 에크린 구조에서 LL-37 단백질과 mRNA가 관찰되었습니다. LL-37은 에크린샘과 땀샘 상피 세포 모두에 국한된 반면, 이전에 설명한 땀의 천연 항생제인 더르시딘은 땀샘 자체에서만 발현되었습니다. 땀 이온 환경에서 다양한 박테리아에 대한 LL-37과 더르시딘의 항균 활성은 합성 펩타이드를 사용한 용액 콜로니 형성 분석과 정상 지원자로부터 얻은 땀에서 입증되었습니다. 이러한 결과는 카테리시딘이 사람의 땀에서 분비되고 그람 양성 및 그람 음성 박테리아 모두에 대해 강력한 항균 활성을 가지며 프리폼에서 처리 후 감염으로부터 보호하는 장벽을 제공할 수 있음을 나타냅니다. 따라서 땀은 염증이 없는 상태에서 강력한 선천 면역 효과 분자를 전달하는 독특한 전달 방식입니다.
Antimicrobial peptides are an important component of the innate response in many species. Here we describe the isolation of the gene Dermcidin, which encodes an antimicrobial peptide that has a broad spectrum of activity and no homology to other known antimicrobial peptides. This protein was specifically and constitutively expressed in the sweat glands, secreted into the sweat and transported to the epidermal surface. In sweat, a proteolytically processed 47–amino acid peptide was generated that showed antimicrobial activity in response to a variety of pathogenic microorganisms. The activity of the peptide was maintained over a broad pH range and in high salt concentrations that resembled the conditions in human sweat. This indicated that sweat plays a role in the regulation of human skin flora through the presence of an antimicrobial peptide. This peptide may help limit infection by potential pathogens in the first few hours following bacterial colonization.
항균 펩타이드는 많은 종에서 선천적 반응의 중요한 구성 요소입니다. 여기에서는 광범위한 활성을 가지며 다른 알려진 항균 펩티드와 상동성이 없는 항균 펩티드를 암호화하는 Dermcidin 유전자의 분리에 대해 설명합니다. 이 단백질은 땀샘에서 특이적이고 구성적으로 발현되어 땀으로 분비되어 표피 표면으로 운반됩니다. 땀에서 다양한 병원성 미생물에 반응하여 항균 활성을 보이는 단백질 분해 처리된 47-아미노산 펩타이드가 생성되었습니다. 이 펩타이드의 활성은 사람의 땀의 조건과 유사한 넓은 pH 범위와 높은 염분 농도에서도 유지되었습니다. 이는 땀이 항균 펩타이드의 존재를 통해 인간의 피부 세균총을 조절하는 데 중요한 역할을 한다는 것을 나타냅니다. 이 펩타이드는 박테리아 군집화 후 처음 몇 시간 동안 잠재적 병원균에 의한 감염을 제한하는 데 도움이 될 수 있습니다.
https://www.ncbi.nlm.nih.gov/pmc/articles/PMC4369929/
Temperature (Austin). 2019; 6(3): 211–259.
Published online 2019 Jul 17. doi: 10.1080/23328940.2019.1632145
PMCID: PMC6773238
PMID: 31608304
Physiology of sweat gland function: The roles of sweating and sweat composition in human health
Author information Article notes Copyright and License information PMC Disclaimer
Associated DataData Citations
ABSTRACT
The purpose of this comprehensive review is to: 1) review the physiology of sweat gland function and mechanisms determining the amount and composition of sweat excreted onto the skin surface; 2) provide an overview of the well-established thermoregulatory functions and adaptive responses of the sweat gland; and 3) discuss the state of evidence for potential non-thermoregulatory roles of sweat in the maintenance and/or perturbation of human health. The role of sweating to eliminate waste products and toxicants seems to be minor compared with other avenues of excretion via the kidneys and gastrointestinal tract; as eccrine glands do not adapt to increase excretion rates either via concentrating sweat or increasing overall sweating rate. Studies suggesting a larger role of sweat glands in clearing waste products or toxicants from the body may be an artifact of methodological issues rather than evidence for selective transport. Furthermore, unlike the renal system, it seems that sweat glands do not conserve water loss or concentrate sweat fluid through vasopressin-mediated water reabsorption. Individuals with high NaCl concentrations in sweat (e.g. cystic fibrosis) have an increased risk of NaCl imbalances during prolonged periods of heavy sweating; however, sweat-induced deficiencies appear to be of minimal risk for trace minerals and vitamins. Additional research is needed to elucidate the potential role of eccrine sweating in skin hydration and microbial defense. Finally, the utility of sweat composition as a biomarker for human physiology is currently limited; as more research is needed to determine potential relations between sweat and blood solute concentrations.
이 포괄적인 검토의 목적은 다음과 같습니다:
1) 땀샘 기능의 생리와 피부 표면으로 배설되는 땀의 양과 성분을 결정하는 메커니즘을 검토하고,
2) 땀샘의 잘 확립된 체온 조절 기능과 적응 반응에 대한 개요를 제공하며,
3) 인체 건강의 유지 및 교란에서 땀의 잠재적인 비체온 조절 역할에 대한 증거 현황을 논의하는 것입니다.
신장과 위장관을 통한 다른 배설 경로에 비해
노폐물과 독성 물질을 제거하는 땀의 역할은 미미한 것으로 보이며,
에크린선은
땀을 농축하거나 전체 발한량을 증가시켜
배설 속도를 높이는 데 적응하지 못하기 때문입니다.
땀샘이
노폐물이나 독성 물질을 체내에서 제거하는 데
더 큰 역할을 한다는 연구는
선택적 수송에 대한 증거라기보다는
방법론적 문제의 산물일 수 있습니다.
또한,
신장 시스템과 달리
땀샘은 바소프레신 매개 수분 재흡수를 통해
수분 손실을 보존하거나
땀액을 농축하지 않는 것으로 보입니다.
땀의 나트륨 염류 농도가 높은 사람(예: 낭포성 섬유증)은
장기간 땀을 많이 흘리는 동안
나트륨 염류 불균형 위험이 증가하지만,
땀으로 인한 미량 미네랄과 비타민 결핍 위험은 미미한 것으로 보입니다.
피부 수분 공급과 미생물 방어에 있어
에크린 땀의 잠재적 역할을 규명하기 위해서는
추가적인 연구가 필요합니다.
마지막으로,
땀과 혈중 용질 농도 사이의 잠재적 관계를 파악하기 위해서는
더 많은 연구가 필요하므로
인체 생리학의 바이오마커로서 땀 성분의 유용성은 현재로서는 제한적입니다.
KEYWORDS: Chloride, potassium, sauna, sodium, sweat biomarkers, thermoregulation
Introduction
Sweat evaporation from the skin surface plays a critical role in human thermoregulation and this is most apparent when the ability to sweat is compromised during periods of strenuous physical labor and/or exposure to hot environments [1]. For example, in anhidrotic patients [2,3] or individuals wearing encapsulating protective clothing/equipment [4], body core temperature rises sharply with exercise-heat stress, which can lead to heat exhaustion or heat stroke if other means of cooling are not provided. Despite the well-accepted thermoregulatory role of sweating, it is common perception that sweating has a variety of other critical homeostatic functions unrelated to thermoregulation. For instance, sweat glands are perceived to play an important excretory function, similar to that of the renal system, responsible for clearing excess micronutrients, metabolic waste, and toxicants from the body. This belief can lead individuals to engage in practices (e.g. prolonged sauna exposure, exercise in uncompensable conditions) designed to induce heavy sweat losses for their perceived health benefits. However, the effectiveness of sweat glands as an excretory organ for homeostatic purposes is currently unclear as there are no comprehensive reviews on this topic. Another common perception is that excretion of certain constituents in sweat may lead to perturbations in health, such as micronutrient imbalances. A few studies have investigated this notion but a thorough review of the literature has not been published to date. Therefore, the first aim of this paper is to provide a comprehensive review of the physiology of sweat gland function, including the types of sweat glands, their structure, and mechanisms that determine the amount and the composition of sweat excreted onto the skin surface. This will provide the background necessary to then discuss the physiological roles of sweat in the maintenance and/or perturbation of human health. In particular, this paper will provide the state of the evidence for the non-thermoregulatory as well as the thermoregulatory roles of sweating, consider the methodological challenges of studies in this area, and make suggestions where future research is needed.
피부 표면에서 땀이 증발하는 것은 사람의 체온 조절에 중요한 역할을 하며, 이는 격렬한 육체 노동이나 더운 환경에 노출되었을 때 땀 배출 능력이 저하될 때 가장 두드러집니다[1]. 예를 들어, 다한증 환자[2,3]나 밀폐형 보호복/장비를 착용한 사람[4]의 경우 운동으로 인한 열 스트레스로 체온이 급격히 상승하여 다른 냉각 수단이 제공되지 않으면 열 탈진이나 열사병으로 이어질 수 있습니다. 땀의 체온 조절 역할은 잘 알려져 있지만, 땀에는 체온 조절과 무관한 다양한 다른 중요한 항상성 기능이 있다는 것이 일반적인 인식입니다.
예를 들어, 땀샘은 신장과 유사한 중요한 배설 기능을 수행하여 과도한 미량 영양소, 대사 노폐물 및 독성 물질을 몸에서 제거하는 역할을 하는 것으로 인식되고 있습니다. 이러한 믿음으로 인해 사람들은 건강에 도움이 된다고 생각하여 땀을 많이 흘리도록 유도하는 행위(예: 장시간 사우나에 노출되거나 보상할 수 없는 조건에서의 운동)를 하게 될 수 있습니다.
그러나 땀샘이 항상성 유지 목적의 배설 기관으로서의 효과는 현재 이에 대한 종합적인 검토가 이루어지지 않아 불분명합니다. 또 다른 일반적인 인식은 땀으로 특정 성분이 배설되면 미량 영양소 불균형과 같은 건강 교란을 초래할 수 있다는 것입니다.
몇몇 연구에서 이 개념을 조사했지만 현재까지 문헌에 대한 철저한 검토는 발표되지 않았습니다. 따라서 이 백서의 첫 번째 목표는 땀샘의 종류, 구조, 피부 표면으로 배출되는 땀의 양과 성분을 결정하는 메커니즘을 포함하여 땀샘 기능의 생리학에 대한 포괄적인 검토를 제공하는 것입니다. 이를 통해 인체 건강을 유지하거나 교란하는 땀의 생리적 역할에 대해 논의하는 데 필요한 배경 지식을 제공합니다. 특히 이 논문에서는 땀의 체온 조절 역할뿐만 아니라 비체온 조절 역할에 대한 증거 현황을 제공하고, 이 분야 연구의 방법론적 과제를 고려하며, 향후 연구가 필요한 부분에 대해 제안할 것입니다.
Types of sweat glands
The purpose of this section is to compare and contrast the three main types of sweat glands: eccrine, apocrine, and apoeccrine [5,6], which are illustrated in Figure 1. Eccrine sweat glands are the most numerous, distributed across nearly the entire body surface area, and responsible for the highest volume of sweat excretion [5]. By contrast, apocrine and apoeccrine glands play a lesser role in overall sweat production as they are limited to specific regions of the body [7–10]. However, it is important to briefly discuss the apocrine and apoeccrine glands since their secretions can also impact the composition of sweat collected at the skin surface.
이 섹션의 목적은 그림 1에 표시된
세 가지 주요 유형의 땀샘인
에크린,
아포크린,
에크린 땀샘은
가장 많고 거의 모든 신체 표면적에 분포하며
가장 많은 양의 땀을 배출합니다[5].
반면
아포크린 땀샘과 아포에크린 땀샘은
신체의 특정 부위에 국한되어 있어 전
체 땀 생성에서 차지하는 역할이 적습니다[7-10].
그러나
아포크린과 아포에크린 땀샘의 분비물은
피부 표면에서 수집된 땀의 구성에도 영향을 미칠 수 있으므로
아포크린과 아포에크린 땀샘에 대해 간략히 설명하는 것이 중요합니다.
Comparison of the apocrine, eccrine, and apoeccrine glands in the axilla.
Eccrine sweat glands
Eccrine glands were the first type of sweat gland discovered; as they were initially described in 1833 by Purkinje and Wendt and in 1834 by Breschet and Roussel de Vouzzeme, but were not named eccrine glands until almost 100 years later by Schiefferdecker [11]. Eccrine glands are often referred to as the small gland variety, but are by far the most ubiquitous type of sweat gland [12]. Humans have ~2–4 million eccrine sweat glands in total and are found on both glabrous (palms, soles) and non-glabrous (hairy) skin [13–15]. Gland density is not uniform across the body surface area. The highest gland densities are on the palms and soles (~250–550 glands/cm2) [16] and respond to emotional as well as thermal stimuli. The density of eccrine glands on non-glabrous skin, such as the face, trunk, and limbs are ~2–5-fold lower than that of glabrous skin [16], but distributed over a much larger surface area and are primarily responsible for thermoregulation.
The eccrine glands are functional early in life and, starting at ~2–3 years of age, the total number of eccrine glands is fixed throughout life [12–14]. Therefore, overall sweat gland density decreases with skin expansion during growth from infancy and is generally inversely proportional to body surface area. As a result, children have higher sweat gland densities than adults [11], and larger or more obese individuals have lower sweat gland densities than their smaller or leaner counterparts [13,17]. However, higher sweat gland density does not necessarily translate to higher sweating rate. In fact, most of the variability in regional and whole-body sweating rate within and between individuals is due to differences in sweat secretion rate per gland, rather than the total number of active sweat glands [18,19]. Eccrine sweat is mostly water and NaCl, but also contains a mixture of many other chemicals originating from the interstitial fluid and the eccrine gland itself. The structure and function of eccrine glands and the composition of eccrine sweat will be discussed in more detail in subsequent sections of this paper.
Apocrine sweat glands
The apocrine gland is a second type of sweat gland, which was first recognized by Krause in 1844 and later named by Schiefferdecker in 1922 [20,21]. Apocrine sweat glands are located primarily in the axilla, breasts, face, scalp, and the perineum [21,22]. As shown in Figure 1, these glands differ from eccrine glands in that they are larger and open into hair follicles instead of onto the skin surface [12]. In addition, although present from birth, the secretory function of apocrine glands does not begin until puberty [23]. Apocrine glands produce viscous, lipid-rich sweat, which is also comprised of proteins, sugars, and ammonia [21,23]. The function of apocrine glands in many species is generally regarded as scent glands involved in production of pheromones (body odor), although this social/sexual function is rudimentary in humans. Apocrine gland innervation is poorly understood, but isolated sweat glands have been found to respond equally to adrenergic and cholinergic stimuli [23].
Apoeccrine sweat glands
A third type of sweat gland, only recently described by Sato et al. in 1987 [23,24] is the apoeccrine gland. Apoeccrine glands develop from eccrine sweat glands between the ages of ~8 to 14 years and increase to as high as 45% of the total axillary glands by age 16–18 [23]. They are intermediate in size, but as the name suggests, apoeccrine glands share properties with both eccrine and apocrine glands. Like apoeccrine glands, apoeccrine glands are limited in distribution, as they are contained to only the axillary region. Apoeccrine glands are more similar to eccrine glands in that the distal duct connects to and empties sweat directly onto skin surface [23]. In addition, the apoeccrine gland produces copious salt water secretions similar to eccrine sweat [23]. The function of this secretion is unknown, but unlikely to play a significant role in thermoregulation since evaporation is inefficient in the axilla region. The innervation of the apocrine gland is still poorly understood, but in vitro models suggest the apocrine gland is more sensitive to cholinergic than adrenergic stimuli [23,24].
Sebaceous glands
Sebaceous glands are not a type of sweat gland but worth mentioning here since their secretions can impact the composition of sweat collected at the skin surface [25]. Sebaceous glands, first described by Eichorn in 1826 [26], are associated with hair follicles and present over much of the body surface but particularly the scalp, forehead, face, and anogenital area [26,27]. They are absent on the palms of hands and soles of the feet [26]. Sebaceous glands are holocrine glands that secrete a viscous, lipid-rich fluid consisting of triglycerides, wax esters, squalene, cholesterol, and cholesterol esters [25–27]. The rate of sebum production is related to the number and size of glands which is under hormonal (androgen) control [26]. The importance of sebaceous gland secretions is uncertain but sebum is thought to have antibacterial and antifungal properties and function as a pheromone [28].
Eccrine glands will be the focus of this review; therefore, unless otherwise specified, sweating rate and sweat composition will hereafter refer to that of the eccrine glands. The reader is referred to other papers for more details on apocrine and apoeccrine glands [12,20–24,27,29,30] as well as sebaceous glands [26–28].
Structure and function of eccrine sweat glands
Anatomy
The anatomical structure of the eccrine sweat gland, illustrated in Figure 2, consists of a secretory coil and duct made up of a simple tubular epithelium. The secretory tubule is continuous with and tightly coiled with the proximal duct. The distal segment of the duct is relatively straight and connects with the acrosyringium in the epidermis [5]. The secretory coil has three types of cells: clear, dark, and myoepithelial. As shown in Figure 2(c), clear cells are responsible for the secretion of primary sweat, which is nearly isotonic with blood plasma [6–8]. The clear cells contain a system of intercellular canaliculi, glycogen, and a large amount of mitochondria and Na-K-ATPase activity [5]. The dark cells are distinguishable by the abundance of dark cell granules in the cytoplasm. Their function is poorly understood, but thought to potentially act as a repository for various bioactive materials involved in regulation of clear cell and duct cell function [9,10]. The function of the myoepithelial cells is provision of structural support for the gland against the hydrostatic pressure generated during sweat production [5]. The duct has two cell layers: basal and luminal cells. Its primary function is reabsorption of Na and Cl ions as sweat flows through the duct, as shown in Figure 2(d). Most of the NaCl reabsorption occurs in the proximal duct, as these cells contain more mitochondria and Na-K-ATPase activity than that of the distal segment of the eccrine duct [5]. The result is a hypotonic final sweat excreted onto the skin surface [6,9].
Structure of the eccrine sweat gland (panels A-B) and mechanisms of sweat secretion in the secretory coil (panel C) and Na and Cl reabsorption in the proximal duct (panel D). ACh; acetylcholine; AQP-5, aquaporin-5; CFTR, cystic fibrosis membrane channel; ENaC, epithelial Na channel; NaCl, sodium chloride.
Mechanisms of secretion and reabsorption
Secretion
The basic mechanism by which secretion of primary sweat occurs in the clear cells, according to the Na-K-2Cl cotransport model, is illustrated in Figure 2(c). First, binding of acetylcholine to muscarinic receptors on the basolateral membrane of the clear cell triggers a release of intracellular Ca stores and an influx of extracellular Ca into cytoplasm. This is followed by an efflux of KCl through Cl channels in the apical membrane and K channels in the basolateral membrane. This leads to cell shrinkage, which triggers an influx of Na, K, and Cl via Na-K-2Cl cotransporters on the basolateral membrane and subsequently Na and K efflux via Na-K-ATPase and K channels on basolateral membrane as well as Cl efflux via Cl channels on apical membrane. Increased Cl concentration in the lumen creates an electrochemical gradient for Na movement across the cell junction [9,10]. In turn, the net KCl efflux from the cell creates an osmotic gradient for water movement into the lumen via aquaporin-5 channels [31–33].
Ion reabsorption
Figure 2(d) shows the mechanism of ion reabsorption according to the modified Ussing leak-pump model. On the apical membrane of the luminal cells passive influx of Na occurs through amiloride-sensitive epithelial Na channels. Active transport of Na across the basolateral membrane of the basal cells occurs via Na-K-ATPase, which is accompanied by passive efflux of K through K channels on the basolateral membrane. The movement of Cl is largely passive via cystic fibrosis membrane channels (CFTR) on both the apical and basolateral membranes [9,34,35]. The two cell layers are thought to be coupled and behave like a syncytium. The sweat duct also reabsorbs bicarbonate, either directly or through hydrogen ion secretion, but the specific mechanism is unknown [5,8,36,37]. The activity of Na-K-ATPase is influenced by the hormonal control of aldosterone [38]. Overall the rate of Na, Cl, and bicarbonate reabsorption is also flow-dependent, such that higher sweating rates are associated with proportionally lower reabsorption rates resulting in higher final sweat electrolyte concentrations [39,40]. This concept will be covered in more detail in the Effect of sweat flow rate section below.
Sweat gland metabolism
Transport of Na across cellular membranes is an active process, thus sweat secretion in the clear cells and Na reabsorption in the duct require ATP. The main route of energy production for sweat gland activity is oxidative phosphorylation of plasma glucose [6,41]. Cellular glycogen is also mobilized in the eccrine sweat gland during sweat secretion, but its absolute amount is too limited to sustain sweat secretion. Thus, the sweat gland depends almost exclusively on exogenous substrates, especially glucose, as its fuel sources [6,42]. Although the sweat gland is capable of utilizing lactate and pyruvate as energy sources, these intermediates are less efficient than glucose [6,9]. Indeed, studies have shown that arterial occlusion of forearms [43,44] and removal of glucose and oxygen from the bathing medium of isolated sweat glands [6,45] inhibits sweat production. Consequently, lactate (as an end product of glycolysis) and NaCl concentrations in sweat rise sharply. Taken together, these results indicate that oxygen supply to the sweat gland is important for maintaining sweat secretion and ion reabsorption [45].
Control of eccrine sweating
Eccrine sweat glands primarily respond to thermal stimuli; particularly increased body core temperature [40], but skin temperature and associated increases in skin blood flow also play a role [9,46–49]. An increase in body temperature is sensed by central and skin thermoreceptors and this information is processed by the preoptic area of the hypothalamus to trigger the sudomotor response. Recent studies suggest that thermoreceptors in the abdominal region [50,51] and muscles [52] also play a role in the control of sweating. Thermal sweating is mediated predominately by sympathetic cholinergic stimulation. Sweat production is stimulated through the release of acetylcholine from nonmyelinated class C sympathetic postganglionic fibers, which binds to muscarinic (subtype 3) receptors on the sweat gland (see Figure 2(c)) [9]. Eccrine glands also secrete sweat in response to adrenergic stimulation, but to a much lesser extent than that of cholinergic stimulation [6,53]. Catecholamines, as well as other neuromodulators, such as vasoactive intestinal peptide, calcitonin gene-related peptide, and nitric oxide, have also been found to play minor roles in the neural stimulation of eccrine sweating [9,54,55]. In addition, eccrine sweat glands respond to non-thermal stimuli related to exercise and are thought to be mediated by feed-forward mechanisms related to central command, the exercise pressor reflex (muscle metabo- and mechanoreceptors), osmoreceptors, and possibly baroreceptors [55,56].
Sweating rate over the whole body is a product of the density of active sweat glands and the secretion rate per gland. Upon stimulation of sweating, the initial response is a rapid increase in sweat gland recruitment, followed by a more gradual increase in sweat secretion per gland [13,57–59]. Two important aspects of thermoregulatory sweating, depicted in Figure 3, are the onset (i.e. body core temperature threshold) and sensitivity (i.e. slope of the relation between sweating rate and the change in body core temperature) of the sweating response to hyperthermia [60]. Shifts in the sweating temperature threshold are thought to be central (hypothalamic) in origin, whereas changes in sensitivity are peripheral (at the level of sweat glands) [61].
An illustration of central and peripheral control of sweating and the factors that modify the sweating response to hyperthermia. Shifts in the onset (threshold) and sensitivity (slope) of the sweating response to hyperthermia are depicted by the dashed lines. Other potential factors that may directly or indirectly modify sweating (altitude/hypoxia, microgravity, menstrual cycle, maturation, aging) are discussed in the text.
Modifiers of eccrine sweating
Several intra- and interindividual factors can modify the control of sweating [60], some of which are shown in Figure 3. For example, the enhancement of sweating with heat acclimation [62–65] and aerobic training [66–69] has been associated with both an earlier onset and greater responsiveness of sweating in relation to body core temperature [64,70–75]. By contrast, dehydration has been shown to delay the sweating response [76,77], as hyperosmolality increases the body temperature threshold for sweating onset [78–81]. Hypovolemia may reduce sweating sensitivity [82], but this finding has not been consistent [79,83].
Other examples of host and external factors that modify regional and/or whole-body sweating are provided in Table 1. For example, older adults exhibit a lower sweat output per activated gland in response to a given pharmacological stimulus or passive heating compared with younger adults [84–86]. This decline in sweating occurs gradually throughout adulthood [85,87] and there are regional differences in the age-related decrement in sweat gland function [88–91]. However, the decline in sweating rate with aging has been primarily attributed to mechanisms related to a decline in aerobic fitness and heat acclimation (possibly due to decreased sensitivity of sweat glands to cholinergic stimulation [67,92]), rather than age per se [67,68,85,93–96]. In addition, lifetime ultraviolet exposure and other environmental factors may have an interactive effect with chronological age in determining sweat gland responsiveness [84]. Nonetheless, it is important to note that most studies have reported no significant difference in sweating between older and younger adults during exercise in the heat; with the exception of peak sweating rates associated with hot dry climates [94,97,98]. Therefore the ability of older adults to maintain body core temperature during heat stress is usually not compromised. When the effects of concurrent factors, such as fitness level, body composition, and chronic disease are removed, thermal tolerance appears to be minimally compromised by age [93].
Table 1.
Host and environmental factors that modify sweat gland function.
TimingEffect on sweating rate and/or sweat composition
Host and external factors | ||
Dietary NaCl | Acute/ Chronic | No effect on sweating rate [224,236,242–244]; mixed results for effect on sweat [Na] and [Cl] (see Table 5): most studies involving several days to weeks of dietary Na manipulation are associated with significant changes in sweat [Na] [224,235,236,239,240], but there is no correlation between the change in Na intake and the change in sweat [Na] [247]; shorter duration (≤3 days) of Na manipulation has minimal or no impact [134,195,242,244,245] |
Dietary intake of other minerals (Ca, Fe, Zn, Cu) and vitamins (ascorbic acid, thiamine) | Acute/ Chronic | No effect on sweat mineral or vitamin concentrations [230,231,248–250] |
Fluid intake | Acute | Water ingestion results in a reflex (oropharyngeal) transient increase in RSR, especially when in a hypohydrated state [360,361]; no effect on sweat Na, K, Cl, and lactate concentrations [361] |
Dehydration | Acute | Reduced WBSR and RSR attributed to hyperosmolality-induced increase in threshold for sweat onset and to a lesser extent by a hypovolemia-induced decrease in sweat sensitivity (see Figure 3) [42,76–78,80,82]; equivocal effects on sweat [Na] and [Cl] [134,153,190–195]; no effect on sweat [K] [190] |
Alcohol | Acute | No effect on sweating rate [331,332]; sweat ethanol concentration increases with ethanol ingestion and rises linearly with increases in blood alcohol concentration [334,335] |
Exercise Intensity | Acute | Increase in WBSR and RSR with increases in exercise intensity [104,362] as metabolic heat production is directly proportional to energy expenditure [201,203]; sweat [Na] and [Cl] increase with increases in exercise intensity because the relative rate of Na and Cl reabsorption is flow dependent [39,159], minimal or no effect on sweat [K] [159], inverse relation between sweating rate and sweat lactate [162] and ammonia concentrations [6,15] (see Table 4), limited data on other sweat constituents |
Environment | Acute | Increase in WBSR and RSR [201,363–368] with increased environmental heat stress (increased air temperature, increased solar radiation, decreased air velocity) at a given workload; suppression of sweating via hidromeiosis with prolonged exposure to humid/still air [366,369–371]. Increase in sweat [Na] and [Cl] with an increase in ambient temperature [158,372] |
Altitude/hypoxia | Acute | Equivocal effects on sweating; reduced sweat sensitivity for RSR [118,119,373], but mixed results for WBSR [374–378]. Limited data on sweat composition. |
Clothing/protective equipment | Acute | Increase in WBSR and RSR because of reduced evaporative and radiant heat loss from covering the skin surface, heavy protective gear can also increase metabolic heat production [4,379,380]; limited data on sweat composition |
Body mass | Chronic | Increased WBSR in individuals with larger body mass because of increased metabolic heat production at a given absolute workload during weight-bearing exercise [381–383] and possibly lower sweating efficiency [384]; limited data on sweat composition |
Heat acclimation | Chronic | Increase in WBSR [64,220,222,385], variable effects at the regional level such that RSR at the limbs (forearm) tend to increase proportionally more than at central sites (chest, back), suggesting a possible preferential redistribution toward the periphery resulting in more uniform sweating across the body [221,222]; reduced sweat [Na] and [Cl] [62,63,158,217,218,220], but no change in other minerals (K, Mg, Ca, Fe, Cu, Zn) [227]. Sudomotor changes due to gland hypertrophy, increased cholinergic and aldosterone sensitivity, and decreased threshold for sweat onset (see Figure 3). |
Aerobic training | Chronic | Increase in WBSR and RSR because of increased cholinergic sensitivity and decreased threshold for sweat onset [66–69] (see Figure 3); limited data for sweat composition |
Sex | Chronic | Higher WBSR and RSR in men because of greater cholinergic responsiveness (see Figure 3) and maximal sweating rate, but only at high evaporative requirements for heat balance [83,99–102]; otherwise higher WBSR often observed in men are related to higher body mass and metabolic heat production, rather than sex per se [104–108]. Minimal differences in sweat [Na], [Cl], and [lactate] due to sex per se [103,134,156,386–388]; limited data on other constituents |
Menstrual cycle | Cyclical | No effect on WBSR [123,126–128], but lower RSR at a given body core temperature (increased threshold and decreased slope) during luteal phase [122–125]; no effect on sweat [Na], [Cl], or [K] [389] |
Circadian Rhythm | Cyclical | Increased sweating threshold in the afternoon (1200–1600 h) vs. early morning (400–530 h) [121,122]; no data on sweat composition |
Race/Ethnicity | Chronic | No inherent race or ethnicity differences in WBSR, RSR, or sweat composition [134,390,391]; but heat habituation, characterized by lower (lower ASGD and SGO) and more efficient sweating (less dripping), occurs in people indigenous to hot or tropical climates [70,392–397] |
Maturation | Progressive change | Lower WBSR and sweat [Na] in pre-pubertal vs. post-pubertal boys [94,115–117] |
Aging | Progressive change | Reduced WBSR and RSR related to decreased SGO associated with decline in aerobic fitness and heat acclimation rather than aging per se [67,85,93,94]; during exercise-heat stress differences more evident for peak sweating rate (e.g. during exercise in hot dry climates) [97]. Limited data on sweat composition, but there seems to be no impact of age per se on sweat [Na] [67]. |
ASGD: activated sweat gland density; RSR: regional sweating rate; SGO: sweat gland output; WBSR:, whole-body sweating rate.
Table 2.
Common methodological issues.
Methodological issueSweat constituents most affectedRecommendation
Skin cleaning/preparation | |||
Cleaning technique | |||
Timing of collection |
| Begin sweat collection after onset of sweating, i.e. 20–90 min, depending on sweating rate and constituent of interest (shorter for NaCl, longer for trace minerals) to allow flushing of contaminants from lumen and time to reach steady state sweating rate, then clean skin/wipe away sweat from surface prior to collection [228,229,234] | |
Sweat stimulation | |||
Sauna (steam) | Contamination from steam condensation on skin [25] | Use collection method (Parafilm-M® pouch, absorbent patch) that prevents contamination from surrounding steam | |
Passive (dry) heat | May not be entirely applicable to sweating response in athletes during exercise (non-thermal stimuli) [55,56] | Potentially all sweat constituents; sweat [Na] and [Cl] lower during passive heat vs. exercise [134,402] | Use if interested in measuring sweat composition in response to environmental heat stress alone (non-athletes). Avoid if interested in understanding sweat constituent losses relevant to exercise [132,137,403,404] |
Exercise | Limited to certain collection methods during exercise, especially in athletes in contact sports [132] | All sweat constituents |
|
Pharmacological (e.g. pilocarpine iontophoresis) | Sweat secretion only induced via local cholinergic stimulation of sweat glands, whereas with exercise and/or heat stress other local and central mediators are involved in sweat stimulation [55,56] | Significantly different RSR response, pH, and sweat constituent concentrations (Na, Cl, K, lactate) compared with exercise/heat stress [8,16,134,404–408] | Appropriate for research regarding physiological mechanisms of local control of sweating. Avoid if interested in understanding sweat constituent losses relevant to exercise/whole body heat stress [132,403,404] |
Sweat collection | |||
Whole body washdown | Primarily limited to laboratory studies | All sweat constituents | Criterion method because all sweat loss is accounted for and normal evaporation is permitted. Whole body washdown is preferred, especially when quantifying total sweat losses or conducting electrolyte/micronutrient balance studies [134,144,403,409] |
Regional methods (in general) | Up to 2–3.5 fold inter-regional variability for sweat Ca, Mg, Zn, Cu, Fe, Na, Cl [146,147,159,274,286]; inter-regional variability minimal for K [146,159] | Collect sweat from regions that are most representative or highly correlated with whole body across various sweating rates (e.g. forearm for [Na] and [Cl]) [159] | |
Absorbent patches |
| ||
Parafilm-M® pouch |
| All sweat constituents | Preferred method for serial measurements of composition (via aspiration of sweat at desired intervals) [413] |
Macroduct®/Megaduct |
| All sweat constituents | May be appropriate for use during prolonged heat exposure (>60 min), when not interested shorter duration exercise or serial measurements [414] |
Ventilated sweat capsule | RSR mostly; not often used to measure sweat composition | Criterion method for RSR; preferred method, especially if maximal RSR representative of compensable environment is desired [417–419] | |
Arm bag | Overestimation (by 1.5-6x) in sweat Na, Cl, K, Mn, nickel, lead, Cu, Fe, Zn, Ca [228,285,420,421] | Avoid or use modified technique excluding the hand [422] | |
Scraping methods | Avoid | ||
Dripping methods | Evaporation of water portion of sweat and surface contamination [399] | Overestimation in all sweat constituent concentrations due to evaporation [399] | Avoid |
Sample storage | |||
Sealing, temperature, duration | Evaporation of water portion of sweat; mold growth | 6–42% increase in sweat [Cl] after 3 days and 12–66% increase after 5 days when vials not Parafilm-M®-sealed [424]. When sealed during storage, no change in sweat [Na], [Cl], or [K] when refrigerated, frozen, or at room temperature for 7 days [425]. | Seal (e.g. Parafilm-M®) in an airtight tube [424]; refrigerate for up to 1–2 weeks [143,425], or freeze when longer storage durations are necessary |
Analytical technique | |||
Laboratory and field |
| Sweat [Na] ion chromatography ≤ ion-selective electrode < flame photometry ≤ conductivity [132,156,372,426–429], 4–30% variation between techniques; not enough information on other sweat constituents |
RSR: regional sweating rate.
Table 3.
Sweat micronutrients: Mechanisms and methodological considerations.
Concentration in sweatComparison of regional and whole body sweatCorrelation between sweat and bloodSweat gland mechanismsPotential methodological Issues
Sodium | 10–90 mmol/L [145–148,156] | Significant correlation; many (forehead, back, chest, upper arm) but not all (foot, calf, thigh) regional sites overestimate whole body concentrations [146,147,149,159] | a [228,295]; plasma [Na] influences sweat [Na], but not reliably correlated because of other factors that dictate reabsorption rates | Secreted via paracellular transport [15]. Primary sweat is nearly isotonic with blood plasma [8,307,434]; Na is reabsorbed in the duct via epithelial Na channels and Na-K-ATPase (activity influenced by aldosterone) [38] transporters [9,34] (see Figure 2), resulting in hypotonic final sweat | Concentration varies (up to 2–3 fold) with sweating rate [6,39,40,159] |
Chloride | 10–90 mmol/L [147–149,152,159,435] | Significant correlation; many (forehead, back, chest, upper arm) but not all (foot, calf, thigh) regional sites overestimate whole body concentrations [146,147,149,159] | a [152,195]; plasma [Cl] influences sweat [Cl], but not reliably correlated because of other factors that dictate reabsorption rates | Secreted via Na-K-2Cl cotransport model [15]. Primary sweat is slightly hypertonic compared with blood plasma [8,434]; Cl is reabsorbed in the duct via the CFTR [35] (see Figure 2), resulting in hypotonic final sweat | Concentration varies (up to 2-3 fold) with sweating rate [6,152,159] |
Potassium | 2–8 mmol/L [146,147,435] | Mixed results with respect to correlation [146,147,149,159] | a [228] | Secreted via Na-K-2Cl cotransport model [15]. Primary sweat is nearly isotonic with blood plasma [6,8,434]; Mixed results with respect to relation between flow rate and sweat [K] [6,147,159,436]; thought to be secreted during sweat passage along the duct, but mechanism unknown [437–439] | Often overestimated (by up to 2-3x) with arm bag technique due to surface contamination [134,228] |
Calcium | b0.2–2.0 mmol/L [144,219,227–229,235,286] | No correlation; regional measures overestimate whole body concentrations [286] | No [283] | NA | Overestimation (by up to 3x) both of epidermal origin and residual Ca in the sweat gland lumen [134,228] |
Magnesium | b0.02–0.40 mmol/L [219,227–229] | No correlation; regional measures overestimate whole body concentrations [286] | NA | NA | Overestimation (by up to 3x) from skin surface contamination [228] |
Iron | b0.0001–0.03 mmol/L [219,228,233,234,274] | Regional measures overestimate whole body concentrations [285] | No [233,251] | NA | Overestimation (by up to 2-3x) both of epidermal origin and residual Fe in the sweat gland lumen [134,228,233,234]; cell-rich sweat particularly high in Fe [184,233,234,253,254] |
Zinc | b0.0001–0.02 mmol/L [219,227–229,274] | Regional measures overestimate whole body concentrations [285] | NA | NA | Overestimation (by up to 2x) from skin surface contamination [228] |
Copper | b0.0005–0.02 mmol/L [219,227–229,274,285,286] | No correlation; regional measures overestimate whole body concentrations [285,286] | NA | NA | Overestimation (by up to 3.5x) from skin surface contamination [228] |
Vitamins | a | NA | NA | NA | Overestimation from skin surface contamination and sebum secretions [25,134] |
NA: no data available; amixed results or too few studies available to draw conclusion; bvalues are from regional or whole-body sweat reported from studies that took measures to prevent epidermal contamination (e.g. pre-rinsed skin and analyzed cell-free sweat)
Table 4.
Selected non-micronutrient components of eccrine sweat: Mechanisms and methodological considerations.
Concentration in sweatComparison of regional and whole body sweatCorrelation between sweat and bloodSweat gland mechanismsFunctional role in eccrine sweatPotential methodological Issues
Lactate | 5–40 mmol/L [6,45,147,162,440–443] | No correlation [147] | No [45,181,440–442] | Produced by eccrine sweat gland metabolism [13,15,45,134,140]. Inverse relation between sweating rate and sweat lactate concentration (dilution effect), but direct relation between sweating rate and lactate excretion rate [161–163]. | Natural skin moisturizer [15,214] Excretion of metabolic waste – not enough evidence [440] | Concentration varies with changes in sweating rate. Skin surface contamination from residual lactate in sweat ducts [163,442] |
Urea | 4–12 mmol/L [45,193,441] | Significant correlation, but regional measures overestimate whole body concentrations [444] | a [337,339,441] | Primarily derived from plasma [239]. Readily crosses glandular wall and cell membrane and therefore concentrations expected to be same as or slightly higher than plasma [15]. However, measured concentrations are often significantly higher in sweat than plasma [337–339]; possibly because synthesis of urea by the gland [6] or surface contamination issues. | Natural skin moisturizer [15] Excretion of metabolic waste – not enough evidence [134] | Concentration changes with variation in sweating rate [6]. Skin surface contamination from residual urea in sweat gland lumen [183,239] |
Ethanol | 2–30 mmol/L [334,335] | NA | Yes [334,335] | Primarily derived from plasma [334,335]. | Detoxification – not enough evidence [336] | Evaporation of ethanol during sweat collection [401] |
Ammonia | 1–8 mmol/L [15,193,400,441] | Regional measures overestimate whole body concentrations [445] | a [400,441] | Concentrations 20-50x that of plasma and is inversely related to sweating rate and pH. Primarily derived from plasma NH3 by nonionic passive diffusion of NH3 to acidic ductal sweat and ionic trapping of NH4 [6,15]. | Excretion of metabolic waste – not enough evidence [134,193] | Skin surface contamination from residual NH3 in sweat gland lumen and/or breakdown of urea by bacteria on skin [400] |
Bicarbonate | 0.5–5.0 mmol/L [147,443] | NA | a [443] | Primary fluid in secretory coil is lower than blood plasma [5,434]. HCO3 is reabsorbed in the sweat duct (via CFTR directly [36] or via H+ secretion), resulting in acidification of final sweat [36]. HCO3 reabsorption is inversely related to sweating rate (i.e. reabsorption is higher at low sweating rate). Thus final sweat pH is lower (more acidic) at lower sweating rate [5,8,37,160] | Dictates pH of sweat [5,8] | Concentration varies with changes in sweating rate [37,160] |
Glucose | 0.01–0.20 mmol/L [183,446,447] | NA | a [134,446–448] | Secreted via paracellular transport [449]. Plasma glucose is the primary energy source for eccrine sweat gland secretory activity [6,41]. | NA specific to its presence in sweat | Possible skin surface contamination from residual glucose in sweat ducts |
Heavy Metals (e.g. arsenic, lead, mercury, cadmium) | Lead 0.00002–0.00006 mmol/L [285,325] | Regional measures overestimate whole body concentrations [285] | No [318,325] | Concentrations are often significantly higher in sweat than plasma [280,314,318], but no known mechanisms for preferential secretion. | Detoxification – not enough evidence [325] | Skin surface contamination from epidermis and/or sebum secretions [318,321,322] |
Antibodies (e.g. IgG, IgA) and Antimicrobial peptides (e.g. dermcidin, cathelicidin, lactoferrin) | a | NA | NA | Mechanism of secretion unclear [450]; produced by eccrine sweat gland [211–214] | Protect against infections by controlling certain pathogenic bacterial counts on skin surface [211–214] | Skin surface contamination from residual antibodies and antimicrobial peptides in sweat ducts |
Other Proteins (e.g. albumin, α-globulin, γ-globulin) | a | NA | NA | Paracellular transport, but pathway not fully understood; thought to involve tight-junction remodeling [451] | NA specific to its presence in sweat | Concentration varies with sweating rate [15]. Potential for contamination by epidermal protein [6]. |
Cytokines (e.g. Interleukin-1α, 1β, 6, 8, 31, TNFα) | a | NA | a [452,453] | Derived from eccrine sweat gland (stress-induced increased secretion of Interluekin-1) and plasma [10,399,454,455]. Concentrations increase with increasing sweating rate [399]. | NA specific to its presence in sweat | Skin surface contamination, both of epidermal origin and residual cytokines in the sweat gland lumen [454] |
Amino acids (e.g. pyrrolidone carboxylic acid, urocanic acid, serine, histadine, ornithine, glycine, alanine, aspartic acid, lysine) | a | NA | NA | Secretory mechanisms are unknown [6]. Present in sweat but varied in concentrations perhaps because of contamination [15] | Natural skin moisturizers, maintain barrier integrity of skin [206,207] | Skin surface contamination, both of epidermal origin and residual amino acids in the sweat gland lumen [15,136,207] |
Proteolytic enzymes | a | NA | NA | Derived from eccrine sweat gland [10,456] and/or epidermis [423]. Concentrations increase with increasing sweating rate [457]. | Skin maintenance and protection via desquamation of horny layer, hydrolysis of debris in the ductal lumen, allergen inhibition [456] | Skin surface contamination, both of epidermal origin [423] and residual proteolytic enzymes in the sweat gland lumen [457] |
Persistent Organic Pollutants (e.g. organochlorinated pesticides, polychlorinated biphenyls, perfluorinated compounds) | a | NA | No [312,316,458] | Concentrations are often significantly higher in sweat than plasma [312,316], but no known mechanisms for preferential secretion. Persistent organic pollutants are lipophilic and thus may appear on skin surface through sebum secretions [458]. | Detoxification – not enough evidence [458] | Skin surface contamination from epidermis and/or sebum secretions [323,458] |
Other toxicants (e.g. BPA, phthalate, polybrominated diphenyl ethers) | a | NA | No [311,313,317] | Concentrations are often significantly higher in sweat than plasma [311,313,317], but no known mechanisms for preferential secretion. | Detoxification – not enough evidence [323,324] | Skin surface contamination from epidermis and/or sebum secretions [323] |
BPA: bisphenol-A; NA: no data available; HCO3: bicarbonate; NH3: ammonia; a mixed results or too few studies available to draw conclusion.
Table 5.
Studies on sodium intake and sweat electrolyte concentration and total electrolyte loss during exercise and/or heat stress.
StudyProtocol/SubjectsStudy ArmsNa and/or Cl intakeDurationSweat [Na] and/or [Cl]Sweating RateSweat Na and/or Cl loss
McCance 1938 [225] | 2 non-HA men; crossover; repeated exposure to passive heat stress; whole body sweat | NaCl deficiency Generous NaCl intake | NA NA | 5–8 days 5–8 days | 72 to 33 mM Na 62 to 70 mM Na | NA NA | 162 to 73 mmol Na 150 to 186 mmol Na |
Weiner and Van Heyningen 1952 [45] | 1 non- HA man; crossover; HA protocol (2 h exercise+heat); whole body sweat | High NaCl followed by Low NaCl | 300 mmol/d (10.7 g/d) Cl 16 mmol/d (0.57 g/d) Cl | 1st week 2nd week | 35 to 40 mM Cl 40 to 13 mM Cl | Increase in WBSR with HA, especially in 1st week: 0.56 vs. 0.85 L/h; end of 2nd week: 0.72 L/h | 40 to 69 mmol Cl 69 to 19 mmol Cl |
Komives et al. 1966 [239] | 4 men (HA status NA); 3–5 h exercise+heat; whole body sweat | Excessive NaCl intake followed by moderate NaCl deficiency | 150–200 mEq (8.8–11.7 g) NaCl over 6 days during salt deficiency | Day 1–7 Day 8–13 | 50 to 15 mM Cl from day 8 to 13 | NC (mean data NA) | NA |
Sigal and Dobson 1968 [240] | 3 men (HA status NA); passive heat stress; forehead sweat via filter paper | High NaCl intake followed by low NaCl intake 1 month later | 20 g/d NaCl 0.5 g/d NaCl | 5 days 5 days | Low NaCl intake associated with lower sweat [Na] at a given sweating rate vs. high NaCl intake (mean data NA). | NC in forehead sweating rate (mean data NA) | NA |
Costa et al. 1969 [235] | 12 HA men; parallel groups (n = 6 each); 40 min exercise in temperate environment; regional sweat via patches and arm bag and whole body sweat | Formula diet Space diet | 3.4 g/d Na 5.6 g/d Na | 6 weeks 6 weeks | Space diet associated with higher sweat [Na] vs. Formula diet:
| ND in WBSR: 0.54 vs. 0.53 L/h | NA |
Costill et al. 1975 [244] | 10 men, 2 women; moderately HA; crossover; 1.5–2.5 h exercise+heat to 3% BML; whole body sweat | Post-exercise rehydration with water or CES, but same daily NaCl intake otherwise | Water CES (1.3% glucose, 23 mM Na, 14.8 mM Cl) | 5 days 5 days | ND between CES and water on day 1, 3, or 5 (mean data NA) | NS between water and CES, but WBSL greater on day 5 than day 1* Day 1: 2.09 vs. 2.09 L Day 3: 2.22 vs. 2.21 L Day 5: 2.30 vs. 2.31 L | No within-day differences between water vs. CES. Increase from Day 1 to Day 5* due to increased WBSR Day 1: 74 vs 73 mmol Na NS Day 3: 71 vs. 78 mmol Na NS Day 5: 84 vs. 88 mmol Na NS |
Konikoff et al. 1986 [242] | 5 HA men; crossover; 2 h exercise+heat; whole body sweat | Control NaCl loading | Normal diet Normal diet+10.2 g/d NaCl tablets | 3 days 3 days | 31 mM Na 26 mM Na NS 21 mM Cl 17 mM Cl** | 0.87 L/h 0.92 L/h NS | NA |
Armstrong et al. 1985 [224] | 9 non-HA men; crossover; HA protocol (1.5 h exercise+heat); whole body sweat | Low Na High Na | 98 mmol/d (2.3 g) Na 399 mmol/d (9.2 g) Na | 8 days 8 days | 45 to 26 mM Na* 41 to 56 mM Na* ** | 0.94 to 0.96 L/h 1.00 to 1.04 L/h NS | 63 to 38 mmol Na* 61 to 87 mmol Na* ** |
Hargreaves et al. 1989 [236] | 8 non-HA men; crossover; 1 h exercise+heat; whole body sweat | Low Na Control | 50 mmol/d (1.15 g/d) Na 150 mmol/d (3.45 g/d) Na | 2 weeks 2 weeks | 38 mM Na at end of 2 weeks 50 mM Na at end of 2 weeks** | 1.12 L/h 1.15 L/h NS | 41 mmol/h Na 55 mmol/h Na** |
Allsopp et al. 1998 [241] | 25 non-HA men; parallel groups; HA protocol (heat only); whole body sweat | Low Na (n = 9) Moderate Na (n = 9) High Na (n = 7) | 66 mmol/d (1.5 g/d) Na 174 mmol/d (4 g/d) Na 348 mmol/d (8 g/d) Na | 5 days 5 days 5 days | NA | NA | 54 to 25 mmol Na* 64 to 39 mmol Na* ** 79 to 53 mmol Na* ** **final sweat Na loss greater in high and moderate groups vs. low group, but groups not matched at baseline |
Koenders et al. 2017 [243] | 9 non-HA men; crossover; 3 h exercise+heat; regional sweat via patches on arm, back, chest, leg | Low Na High Na | Subjects instructed to reduce Na intake Subjects instructed to eat a normal Western diet Compliance assessed via 24h urinary Na excretion: Low Na: 58.6 mmol/d (1.3 g/d) Na High Na: 165.1 mmol/d (3.8 g/d) Na | 9 days 9 days | 55 mM Na 55 mM Na NS (average of 4 sites) | 1.30 L/h 1.24 L/h NS | 74 mmol/h Na 69 mmol/h Na NS |
Robinson et al. 1956 [195] | 4 HA men; 4 x 25-h intermittent exercise+heat protocols (separated by 1 week); whole body sweat | 4 arms in which water and NaCl balanced were controlled |
| 25 h each arm |
| 15% lower in DEH trials; no effect of NaCl balance (mean data NA) | Mean data NA, but hourly sweat Cl output decreased during EUH/Cl depleted trial and increased during DEH/Cl maintained trial |
Hamouti et al. 2012 [245] | 10 non-HA men; crossover; controlled Na intake 1.5h before 2 h exercise+heat; regional sweat via patch on back and [Na] extrapolated to whole body | Control Moderate Na High Na | 0 g Na 1.45 g Na 2.90 g Na | 3.5 h 3.5 h 3.5 h | NS among trials: 36 mM Na 39 mM Na 38 mM Na | NS among trials: WBSR: 1.4 L/h all trials; Back sweating rate: 1.8, 1.9, and 1.8 mg/cm2/min | NS among trials: 110 mmol Na 116 mmol Na 113 mmol Na |
Values are means; *p < 0.05 vs. baseline, **p < 0.05 between Na intake levels; NS: no statistically significant difference. Statistical analysis not reported in refs [45,195,225,235,239,240]. Rows shaded gray involved acute NaCl intake differences before/during exercise. All other studies involved differences in chronic NaCl dietary intake. BML: body mass loss through dehydration; CES: carbohydrate-electrolyte solution; Cl: chloride; DEH: dehydration; EUH: euhydration; HA: heat-acclimated or heat acclimation; NA: not available; NC: no change from baseline (based on authors’ conclusions, but data/stats NA); ND: no difference between Na intake levels (based on authors’ conclusions, but data/stats NA); Na: sodium; NaCl: salt (sodium chloride); SL: sweat loss; WBSR: whole-body sweating rate.
It is often reported that men exhibit higher sweating rates than women; and several factors, some of which are independent effects of sex and others due to confounding physical characteristics, seem to contribute depending upon the study design. Men have a greater cholinergic responsiveness (see Figure 3) and maximal sweating rate than women [83,99–101]. However, studies in which subjects were matched for body mass, surface area, and metabolic heat production, have shown that sex differences in whole-body sweat production are only evident above a certain combination of environmental conditions (e.g. 35–40°C, 12% rh) and rate of metabolic heat production (e.g. 300–500 W/m2) leading to high evaporative requirements for heat balance [83,100–102]. Sweat gland density is generally higher in women than men (due in part to lower body surface area) [17,69,103]. Accordingly, the lower sweating rates by women reported in some studies were a result of lower output per gland [99,101,103]. Otherwise, higher whole-body sweating rates observed in men than women (e.g. in cross-sectional studies) can usually be attributed to higher body mass and metabolic heat production (higher absolute exercise intensities), rather than sex per se [104–109]. Taken together it seems that women are not at a thermoregulatory disadvantage compared with men for most activities and environmental conditions typically encountered [110,111]. As discussed in more detail elsewhere [109,110,112], other factors such as body size, surface area-to-mass ratio, heat acclimation status, aerobic capacity, exercise intensity, and environmental conditions (all of which directly or indirectly impact the evaporative requirement for heat balance) are more important than sex in determining sudomotor responses to exercise-heat stress. The reader is referred to published reviews for more comprehensive discussions on the effects of sex and sex hormones on thermoregulation [110,113,114].
Additional factors, such as maturation [94,115–117], altitude/hypoxia [118–120], circadian rhythm [121,122], and menstrual cycle [122–125] have been shown to modify the onset and/or sensitivity of the sudomotor response (see Table 1). However, modifications in the onset and/or sensitivity of regional sweating in relation to body core temperature are not necessarily associated with significant differences in overall whole-body sweat losses during exercise. Two examples of this were noted above, with respect to the impact of sex and chronological age on sweating. Another example is the menstrual cycle: during the luteal phase regional sweating rate is lower at a given body core temperature (increased threshold and decreased slope) [122–125], but there are no differences in whole-body sweating rate across the menstrual cycle phases [123,126–129]. Additionally, for trained females their menstrual phase is of little physiological or performance consequence during exercise in the heat [103,130].
Some of the variability in sweating rate can be explained by differences in the structure of sweat glands. For example, with habitual activation, sweat glands show some plasticity in their size and neural/hormonal sensitivity [18,19]. Sato and colleagues have shown that glandular size (volume) can vary by as much as fivefold between individuals [9,131], and there is a significant positive correlation between the size of isolated sweat glands and their methacholine sensitivity and maximal secretory rate [131] (see Figure 4). Sweat gland hypertrophy and increased cholinergic sensitivity have been reported to occur with aerobic training [131] and heat acclimation [38] (see Table 1 for more information).
Top row (panels A-C): Variation in the size of human eccrine sweat glands taken from the backs of three different men who were described as poor (A), moderate (B), and heavy sweaters (C). Bottom row: Correlation between size of sweat gland and sweat ratemax per gland (panel D). Dose-response curves (expressed per unit length of tubule) of sweat rates of 7 men to methacholine. Closed symbols show moderate to heavy sweaters. Open symbols show poor sweaters. Reprinted from Sato and Sato 1983 [131]with permission.
Eccrine sweat composition
Methodological considerations
In science, the accuracy and reliability of study methodology are critical to interpret results and draw conclusions about the impact of an intervention or other factor on the outcome measure of interest. Measuring sweat composition is no exception. Previous papers have comprehensively reviewed the effect of methodology on intra- and inter-individual variability in sweating rate and sweat composition as well as made suggestions for best practices [16,83,132]. Therefore, this topic will not be reviewed extensively here. Instead, these methodological considerations and supporting references have been summarized in Table 2. This table includes the main aspects of sweat methodology that are important to consider when interpreting and designing sweat composition research; these include 1) skin cleaning/preparation, 2) sweat stimulation, 3) sweat collection, 4) sample storage, and 5) analytical technique.
Specific examples illustrating the importance of valid methodology in interpreting study results are discussed throughout the remaining sections of this paper. However, it is worth noting a couple of overarching themes. First, it is important to realize that depending upon the methodology used, sweat collected from the surface of the skin may contain, not only thermal sweat secreted by the eccrine sweat gland but also, residual contents of the sweat duct, sebum secretions, epidermal cells, and other skin surface contaminants. This can lead to artificial elevations in sweat constituent concentrations and, in some cases, the overestimation is not small. For example, as shown in Table 2, two- to five-fold increases in constituent concentrations have been reported for trace minerals such as Fe and Ca. Unless care is taken to avoid contaminants it is difficult to draw conclusions about sweat composition and its utility as a biomarker, its impact on micronutrient balance, and assess the effectiveness of the sweat gland in excretion of waste products or toxicants. By contrast, dermal contamination from extra-sweat NaCl seems to be negligible compared with NaCl contained in the sweat itself, as studies have reported only 0.2 mmol/h of Cl on the skin without sweat activity [133–135].
Another important methodological consideration is to ensure that the conditions of the protocol, including the method of sweat stimulation and anatomical location of sweat collection, are specific to the research question of interest. As described in Table 2 sudomotor responses vary among pharmacological-, passive heat-, and exercise-induced sweating and so these methods should not be used interchangeably. Similarly, because of regional variability in sweating rate and sweat constituent concentrations, data from one region cannot be generalized to other regions or the whole body.
Overview of sweat composition
Sweat is a very complex aqueous mixture of chemicals. Although sweat is mostly water and NaCl, it also contains a multitude of other solutes in varying concentrations [6,136–139]. Tables 3 and 4 list some of the micronutrients and non-micronutrients, respectively, present in sweat. This is obviously not an exhaustive list but includes some of the more commonly researched constituents. Tables 3 and 4 include the range in sweat constituent concentrations, mechanisms of secretion and reabsorption, and functional role in health, where known or applicable. Micronutrients include the electrolytes Na and Cl, which are the constituents found in the highest concentrations in sweat, as well as K, vitamins, and trace minerals. Non-micronutrient ingredients listed in Table 4 include products of metabolism, proteins, amino acids, and toxicants. It is important to note that concentrations listed in these tables are approximate ranges and are not intended to reflect normal reference ranges. There are insufficient data, perhaps with the exception of Na, Cl, and K, to inform normative ranges for sweat constituents at this time. Instead the ranges listed are meant to provide some context in terms of relative order of magnitude of concentrations across all of the constituents, in order of higher (e.g. NaCl) to lower (e.g. trace minerals and heavy metals) concentrations (in mmol/L). For some constituents, higher values outside the range listed have been reported, but are relatively rare, involve individuals with medical conditions, or may be inflated because of methodological issues; all of these points are discussed in more detail in later sections of this paper.
Because interstitial fluid is the precursor fluid for primary sweat, it follows that many components of final sweat originate from this fluid space. However, the exact mechanisms of secretion are largely unknown for most constituents other than Na and Cl. Potential mechanisms and supporting references are listed in Tables 3 and 4 and may include active or passive (diffusion across membranes or paracellular transport) mechanisms of transport. Some sweat constituents do not originate from the interstitial fluid, but instead, appear in sweat as a result of sweat gland metabolism (e.g. lactate) [140]. Yet others (e.g. antimicrobial peptides, proteolytic enzymes) are thought to be produced by the sweat gland and play a functional role in skin health (Table 4). It should be noted that many other chemicals (not in Tables 3 and 4), such as cortisol [141,142] neuropeptides, bradykinin, cyclic AMP, angiotensins, and histamines [9,15] are also present in sweat. Some researchers have hypothesized that one or more of these ingredients may be biologically functional, and involved in the regulation of sweat gland and/or ductal function; however, support for this notion is currently limited [9]. For a more comprehensive list of sweat constituents, the reader is referred to other published reviews [6,134] and studies [139,143], including metabolomic analysis of sweat [136–138].
Sodium chloride
It is well established that sweat [Na] and [Cl] can vary considerably among individuals. Regional sweat [Na] typically ranges from 10 to 90 mmol/L (Figure 5(a); see also [144–148]), while whole-body sweat [Na] is ~20–80 mmol/L (predicted shown in Figure 5(b); measured in references [144,146,147,149]). The range in sweat [Cl] is similar, but perhaps slightly lower than that of sweat [Na], with whole-body values reported to be ~20–70 mmol/L [134,144,149]. Table 1 shows the host and environmental factors that account for some of the variability in sweat [Na] and [Cl]. The [Na] and [Cl] of final sweat are determined predominately by the rate of Na reabsorption in the duct relative to the rate of Na secretion in the clear cells.
Frequency histograms of forearm sweat sodium concentration (Panel A) and predicted whole-body sweat sodium concentration (Panel B) in 506 skill-sport and endurance athletes during training/competition in a wide range of environmental conditions. The vertical line represents the mean value. Reprinted from Baker et al. 2016 [156] with permission.
Na ion reabsorption is controlled by Na-K-ATPase activity, which is influenced by plasma aldosterone concentration and/or sweat gland sensitivity to aldosterone. Resting (genomic) plasma aldosterone is dictated by an individual’s chronic physiological condition, dictated in part by heat acclimation, fitness, and diet. Circulating aldosterone also changes acutely in response to non-genomic factors such as exercise and dehydration. Yoshida et al. [150] demonstrated that individual variations in the sweat [Na] response to an increase in the sweating rate during exercise were correlated with resting aldosterone, but not to exercising aldosterone. Therefore the genomic action of aldosterone may have a stronger impact on inter-individual variations in sweat [Na] than the rapid non-genomic action of aldosterone during exercise in humans [150]. Both [Na] and [Cl] in sweat are influenced by the availability of CFTR chloride channels; with lower CFTR abundance resulting in less ductal reabsorption and therefore higher final sweat [Na] and [Cl]. CFTR availability is clearly reduced with defects in CFTR genes (i.e. cystic fibrosis, discussed in more detail in the Sweat composition as a biomarker section below) and recent evidence suggests that healthy (non-CF) individuals with salty sweat may also exhibit lower abundance of sweat duct Cl channel CFTR [151].
Effect of sweat flow rate
Sodium chloride
Sweat flow rate is another important factor determining final sweat [Na] and [Cl] and of other aspects of sweat composition. This concept has been known since as early as 1911 [152] and several studies since then have confirmed a direct relation between sweating rate and final sweat [Na] and [Cl] [5,6,11,15,40,153,154]. In 2008, Buono et al. [39] reported data providing insight to the physiological mechanism responsible for the relation between sweat flow rate and sweat [Na] and [Cl]. They found that as forearm sweating rate increased (from ~0.25 to 0.82 mg/cm2/min), the rate of Na secretion in primary sweat increased proportionally more than the rate of Na reabsorption along the duct [39]. Within this range in sweating rate, which was stimulated via a progressive increase in exercise intensity (from 50% to 90% HRmax), sweat [Na] increased from 19 ± 5 to 59 ± 10 mmol/L (Figure 6) [39]. An important point is that the absolute rate of Na reabsorption actually increased continuously with increases in sweating rate. However, the percentage of secreted Na that was reabsorbed in the duct decreased with a rise in sweating rate. That is, at the lowest sweating rate 86 ± 3% of the secreted Na was reabsorbed, while at the highest sweating rate only 65 ± 6% of Na was reabsorbed from the duct. Therefore, the faster the primary sweat travels along the duct the smaller the percentage of Na that can be reabsorbed [39]. Underlying mechanisms are unclear, but Buono et al. [39] speculated that possible factors could include decreased contact time of sweat with the apical membrane of the duct, saturation of transporters, and/or decreased activity of epithelial sodium channels due to decreased cytosolic pH associated with higher sweating rates. According to some studies, there may be a minimum threshold sweating rate (~0.3 mg/cm2/min) required before sweat [Na] starts to rise with an increase in sweating rate [15,155]. For context, this equates to ~0.3 L/h (for a 1.8 m2 individual), which is at the very low end of the range of sweating rates expected during exercise/heat stress [105,156,157].
Relation between regional sweating rate and regional sweat [Na]. Values are means ± SE for 10 subjects’ regional (forearm) sweating rate and sweat [Na] while exercising at 50%, 60%, 70%, 80%, and 90% of maximal heart rate. The mean r for the group was 0.73 (P < 0.05). y = 59.7(x)+6.7. Reprinted from Buono et al. 2008 [39] with permission.
Given the well-established relation between sweat flow rate and sweat electrolyte concentrations, it follows that any factors stimulating acute increases in sweating rate (e.g. increases in air temperature or exercise intensity) within an individual would result in higher sweat [Na] and [Cl] [152,158]. This has been found at the whole-body level [159] (Figure 7) as well as within isolated sweat glands [6] and given skin regions [39]. The effect of sweat flow rate on relative Na reabsorption may also partially explain regional differences in sweat [Na] and [Cl] within subjects. Studies measuring sweating rate and sweat [Na] and/or [Cl] across multiple body sites have found that sites with higher sweating rate also tend to exhibit higher sweat [Na] and [Cl] [146,147]. This concept is illustrated in Figure 8, which shows a significant correlation (r = 0.71, p < 0.05) between mean regional sweating rate and mean sweat [Na] across eight different regions, where sweat [Na] ranged from 36 mmol/L on the calf (lowest sweating rate) to 72 mmol/L on the scapula (highest sweating rate) [149].
Whole-body sweating rate and whole-body sweat [Na] and [Cl] comparison between low (45% maximal oxygen uptake) and moderate (65% maximal oxygen uptake) intensity cycling exercise in a warm (30°C and 44% relative humidity) environment (n = 11 men and women). Solid circles show individual data. Open circles show mean data (p < 0.05 between low and moderate intensity for sweating rate, sweat [Na] and sweat [Cl]). Redrawn from Baker et al. 2019 [159].
Regional sweating rate vs. regional sweat [Na]. Data points represent the group (26 subjects) mean ± SEM at each regional site (DFA, dorsal forearm; VFA, ventral forearm). Regional sweating rate and sweat [Na] measured with the absorbent patch technique during cycling exercise in the heat (30°C, 42% relative humidity). Redrawn from Baker et al. 2018 [149].
To date, the relation between sweat flow rate and sweat [Na] has been well-established in studies in which subjects served as their own control (e.g. Figure 6–8). However, the regional sweating rate vs. regional sweat [Na] relation for between-subject comparisons has been researched to a lesser extent. When plotting regional sweating rate vs. regional sweat [Na] across subjects, Baker et al. [149] found a significant relation between sweating rate and sweat [Na] at only one region (thigh, r = 0.43) out of 11 regions studied and no significance at the whole-body level (Figure 9). This may suggest that other factors affecting sweat [Na] and [Cl], such as CFTR genes or genomic effects of aldosterone on Na-K-ATPase may play a more important role in determining inter-individual differences in sweat [Na] and [Cl] during exercise/heat stress. On the other hand, acute changes in sweating rate play a significant role in intra-individual differences in sweat [Na] and [Cl] [39,159].
Regression of regional sweating rate vs. regional sweat [Na] within site for the dorsal forearm (A), and the 9-site aggregate (weighted for body surface area and regional sweating rate), as well as regression of whole-body sweating rate vs. whole-body sweat [Na]. Correlations between sweating rate and sweat [Na] were not significant (p > 0.05). Reprinted from Baker et al. 2018 [149] with permission.
Bicarbonate, pH, and lactate
In addition to Na and Cl conservation, another important function of the sweat gland is reabsorption of bicarbonate for the maintenance of acid-base balance of the blood [8]. Exact mechanisms are not fully understood, but it is thought that bicarbonate is reabsorbed directly via CFTR chloride channels [36] and/or hydrogen ions are secreted in the sweat duct [5]. In the process, sweat fluid in the ductal lumen is acidified before excretion onto the skin surface [36]. The pH of primary sweat starts at ~7.1–7.4 [5,8]. Bicarbonate reabsorption in the duct is inversely related to sweating rate [5,8,37,160]. At low sweating rates, the luminal fluid is exposed to the duct for a longer period of time and is acidified to a greater extent, resulting in a decrease in pH to as low as ~4–5 [5,134]. At faster sweat flow rates, pH of sweat can remain as high as ~6.9 [5,134].
As discussed previously, lactate is produced by eccrine sweat gland metabolism [13,15,45,134,140]. Thus, there is a direct relation between sweating rate and lactate excretion rate, such that the higher the sweating rate (and the greater metabolic activity of the sweat gland) the more lactate is secreted in sweat in terms of mmol/min. However, because of the diluting effect of higher sweat fluid volume, there is an inverse relation between sweating rate and sweat lactate concentration [161–163]. Accordingly, sweat lactate concentration decreases with increasing exercise intensity [161,162]. More details regarding the effect of sweat flow rate on sweat composition are provided in Tables 3 and 4.
Sweat composition as a biomarker
There has been considerable interest recently in the use of sweat as a non-invasive alternative to blood analysis to provide insights to human physiology, health, and performance. The development of wearable devices and sensing techniques for sweat diagnostics is an expanding field. Perhaps the best example of a sweat biomarker is the use of sweat [Cl] for the diagnosis of cystic fibrosis, although this practice is not new [164]. The association between high sweat Cl and cystic fibrosis was first recognized by di Sant’Agnese et al. in 1953 [165]; and subsequently, a standardized sweat test (Quantitative Pilocarpine Iontophoretic Test) was developed by Gibson and Cooke in 1959 [166]. Individuals with cystic fibrosis have higher than normal sweat [Cl] because of a genetic absence of a functioning CFTR (two defective genes, homozygote) [167–170]. The cutoff for a positive sweat test consistent with cystic fibrosis is sweat [Cl] >60 mmol/L [171]. However, sweat [Cl] in cystic fibrosis patients can be much higher, with values in the 80–130 range commonly reported [165,166,172–176]. Because the epithelial Na channels depend upon a functioning CFTR, Na is also poorly reabsorbed in individuals with cystic fibrosis [170]. Individuals with one defective gene for CFTR (heterozygote) may also have elevated sweat [Na] and [Cl] [169,177]. For more details, the reader is referred to the following reviews on cystic fibrosis [169,178–180].
Apart from the use of sweat [Cl] for the diagnosis of cystic fibrosis, the application of sweat diagnostics has been limited to date [181,182]. There are perhaps a few constituents in sweat whose concentrations may change in accordance with large disturbances in homeostasis. For example, sweat glucose concentration has been shown to increase 2–3x in response to oral and intravenous glucose which increased blood glucose concentration to 200–250 mg/dl [183]. In addition, iron-deficient anemic patients have lower than normal [Fe] in sweat (especially in cell-rich sweat [184]) and sweat [Fe] has been shown to increase with iron therapy [185]. However, the utility of glucose, micronutrients, and other constituents as sweat biomarkers is questionable, especially as a real-time monitoring tool, because correlations between sweat and blood have not been established. As shown in Tables 3 and 4, the literature has reported mixed results regarding the correlation between sweat and blood for glucose, cytokines, urea, ammonia, and bicarbonate and no significant correlation for micronutrients, lactate, heavy metals, or environmental toxicants.
One of the proposed uses of sweat composition as a biomarker is the prediction of hydration status from sweat electrolyte concentrations or some ratio of [Na], [Cl], and/or [K] [186–189]. However, a fundamental issue with this assertion is that sweat [Na] and [Cl] are known to vary considerably within and among individuals; and a change in hydration status is only one of many factors that could play a role in this variability [132]. This is further complicated by the fact that dehydration could have differential effects on sweat [Na] and [Cl]. Dehydration-induced hemoconcentration would increase extracellular [Na] and in turn increase Na of the primary sweat, in theory leading to a small increase in final sweat [Na]. On the other hand, dehydration would also be expected to reduce sweating rate (Figure 3), which would, in turn, lead to lower sweat [Na]. Other factors such as heat acclimatization, exercise intensity, environment, diet, and sweat stimulation/collection methodology influence sweat [Na] and [Cl] (Table 1). These confounding factors likely explain the discrepancy in results across studies measuring sweat composition and changes in hydration status. Dehydration has been associated with increased [134,153,190], decreased [191,192], or no change [193–195] in sweat [Na] and [Cl]. Sweat [K] and pH are also poor indicators of hydration status [190]. Additionally, sweat composition (Na, Cl, K, pH, lactate) explains very little of the variation in individuals’ sweating rate during exercise [147,149].
In summary, while the notion of a non-invasive tool for real-time hydration, nutrition, and health monitoring is attractive, more research is needed to determine the utility of sweat composition as a biomarker for human physiological status. To date, few well-designed, adequately powered studies have investigated the correlation between sweat and blood solute concentrations. Moreover, as discussed throughout this paper, final sweat composition is not only influenced by blood solute concentrations, but also the method of sweat stimulation (active vs. passive), ion secretion and/or reabsorption in the proximal duct, sweat flow rate, byproducts of sweat gland metabolism, skin surface contamination from epidermal cells as well as sebum secretions, among other factors. These challenges need to be considered in future research and applications of sweat diagnostics.
Physiological purpose of sweating: Roles in the maintenance/disturbance of human health
Thermoregulation
It is well-established that the primary physiological function of sweating is heat dissipation for body temperature regulation. The mechanical efficiency of humans is ≤30% [196]; therefore, during exercise, a large amount of heat is produced by the contracting muscles as a byproduct of metabolism. In addition, heat is transferred from the air to the body when ambient temperature is greater than skin temperature. With sweating, heat is transferred from the body to water on the surface of the skin. The latent heat of vaporization of sweat is 580 kcal of heat per 1 kg of evaporated sweat (2426 J per gram of sweat) [197]. According to heat-balance theory, the amount of sweat production is determined by the relation between the evaporative requirement for heat balance (Ereq) and maximum evaporative capacity of the environment [198,199]. Ereq is represented by the following equation [200]:
Ereq=M−W±(R+C+K)
where M is metabolic energy expenditure, W is external work, R is radiant heat exchange, C is convective heat exchange, and K is conductive heat exchange [201,202]. The primary means by which the body gains heat is from metabolism (which is directly proportional to exercise intensity) and the environment; therefore, these factors are also the primary determinants of sudomotor activity [201,203]. It is important to note that some sweat can drip from the body and not be evaporated. Therefore during conditions of low sweat efficiency (e.g. humid environment), a higher sweating rate than calculated from Ereq may be needed to achieve a given level of evaporation [198,204]. For a more comprehensive discussion on the role of sweat evaporation in human thermoregulation, the reader is referred to other reviews [83,200,205].
Skin health
Eccrine sweat is thought to play a role in epidermal barrier homeostasis through its delivery of water, natural moisturizing factors, and antimicrobial peptides to the skin surface. Natural moisturizing factors include amino acids (or their derivatives), lactate, urea, Na, and K; which can act as humectants allowing the outermost layers of the stratum corneum to remain hydrated [206]. Some of these natural moisturizing factors, such as lactate, urea, Na, and K originate from eccrine sweat [206], while amino acids on the skin surface may be produced in the stratum corneum [207]. Nevertheless studies have shown that perspiration increases stratum corneum hydration [206,208] and this may occur via moisture transfer from the eccrine gland coil directly into the skin before commencement of surface sweating [13,208]. Therefore, it has been proposed that preservation of sweating may be an important therapeutic strategy for improving atopic dermatitis or other conditions of dry skin [206,209], albeit direct evidence is still needed. On a related topic, wetting of the skin with eccrine sweat on the palmar surfaces can improve tactile sense and enhance grip as an aspect of the fight or flight response in humans [210]. Finally, recent immunohistochemistry studies suggest that sweat glands produce and excrete antimicrobial peptides such as dermcidin [211], cathelicidin [212], and lactoferrin [213], pointing to a potential role of sweating in host defense against skin infection [214]. The reader is referred to recent reviews for more details on the role of sweat in skin hydration [209,214,215] and microbial defense [216].
Role in micronutrient balance
Sweat gland adjustments in response to deficiency or excess
Heat acclimation
Sodium chloride
The changes in sweat [Na] and [Cl] during heat acclimation have been well established and reviewed in previous papers [134,169] and therefore will not be comprehensively discussed here. In brief, adaptation to the heat leads to improved salt conservation through a decrease in sweat [Na] and [Cl] [62,63,152,158,217–220]. While the degree of conservation varies across studies due in part to methodological differences, the reported decrease in sweat [Na] and [Cl] after ~10 days of heat acclimation ranges from ~30% to 60%. Most studies have involved a 7–10-day heat acclimation protocol, but Buono et al. [217] recently showed that Na conservation may begin after just two consecutive days of heat exposure and sweat [Na] decreases linearly over time.
Somewhat paradoxically, the decrease in sweat [Na] and [Cl] occurs despite increases in sweating rate that accompany heat acclimation. This can be explained by the disparate effects of acute changes in sweat flow rate (discussed above) versus the longer-term adaptations in the sweat gland that occur with heat acclimation. Buono et al. [218] found that the linear relation between sweat flow rate (up to 1 mg/cm2/min) and sweat [Na] persist after a 10-day heat acclimation protocol, but there is a downward shift such that the y-intercept of the relation decreased by 15 mmol/L. The slope of the relation did not change after heat acclimation. Thus, at any given sweating rate on the forearm, heat acclimation resulted in significantly lower forearm sweat [Na] [218]. However, changes in the slope and y-intercept in response to heat acclimation have not been established for the relation between whole-body sweating rate and whole-body sweat [Na] or [Cl]. Most heat acclimation studies have measured regional sweat electrolyte concentrations. Because of the variable effects of heat acclimation on regional sweating rate, such that regional sweating rate on the limbs (forearm) tend to increase proportionally more than at central sites (chest, back) [221,222], future research is needed to confirm the effects of heat acclimation on whole-body sweat [Na] and [Cl] and its relation with whole-body sweating rate.
The underlying mechanism for NaCl conservation is thought to be related to increased sensitivity of the sweat gland to circulating aldosterone [62]. Aldosterone impacts Na reabsorption in the eccrine sweat duct by increasing Na-K-ATPase activity [38,223]. However, it is important to clarify that the presence of a salt deficit is required for NaCl conservation to occur with heat acclimation. In studies where subjects consumed enough NaCl to replace losses incurred during the repeated exercise-heat stress, sweat [Na] and [Cl] did not change or increased slightly [45,169,224,225]. This topic will be discussed further in the Diet – Sodium Chloride section below.
Trace minerals
A common question on the topic of heat acclimation is whether or not electrolytes or minerals other than NaCl are conserved. Only a few studies have investigated this and mixed results have been reported. For example, in a study with college basketball players, dermal Ca losses measured via a cotton shirt method decreased by 32% from the first to last day of a 10-day training session [226]. In 2008, Chinevere et al. [219] measured sweat mineral concentrations (Ca, Mg, Fe, Zn, and Cu) using the polyethylene arm glove technique and found a 23–75% decrease in mineral concentrations from day 1 to 10 of a heat acclimation protocol. However, in a subsequent heat acclimation study from the same laboratory, Ely et al. [227] found that the changes in sweat mineral concentrations varied depending upon the sweat collection methodology. In arm bag sweat, [Ca], [Mg], and [Cu] trended progressively downward by 26–29% from day 1 to 10 [227]. However, there were no changes in sweat mineral concentrations with heat acclimation at the scapular site that had been thoroughly washed [227]. The authors attributed the decline in sweat mineral concentrations in this [227] and their previous heat acclimation study [219] to an artifact of epidermal contamination when using the arm bag technique and/or not pre-washing/cleaning the skin at the site of collection [228]. That is, progressive flushing of mineral residue lying on the skin surface with daily-repeated profuse sweating may have contributed to the decrease in sweat mineral concentrations over the 10 days of testing [227].
There have been some suggestions that conservation of sweat trace mineral loss occurs on an acute basis during a single bout of exercise. For example, several studies have shown decreases in sweat mineral (Fe, Zn, Mg, Ca) concentrations during 1–7 h of exercise [229–232]. Because sweat mineral concentrations decreased despite stable or increasing sweating rates over time, it was hypothesized that mineral conservation may have been taking place. However, again, this is likely an artifact of skin surface contamination, as studies using methodology to collect clean or cell-free sweat have shown no evidence of trace mineral conservation in response to acute exercise-induced sweating [228,233,234]. Moreover, there are no known physiological mechanisms by which Ca, Mg, Fe, Cu, and other trace minerals would be reabsorbed by the eccrine sweat gland duct in order to facilitate conservation of loss via sweating.
Diet
Sodium chloride
It is a common perception that Na ingestion influences sweat [Na] or the rate of sweat Na excretion. However, study results to date have been mixed. For example, in a systematic review of six endurance exercise studies, McCubbin and Costa (2018) found no relation between the change in Na intake and the change in sweat [Na] across studies. For example, in one study Costa et al. [235] found just a 4 mmol/L mean difference in whole-body sweat [Na] in men after 6-weeks of consuming either 3.4 g Na/day or 5.6 g Na/day (2.2 g/day intake difference). On the other hand, Hargreaves et al. [236] reported a 12 mmol/L difference in whole-body sweat [Na] after 2 weeks of either 1.15 g Na/day or 3.45 g Na/day (2.3 g/day intake difference). Thus, McCubbin and Costa concluded that the impact of dietary Na intake on sweat [Na] during exercise is uncertain and future studies are needed [237].
Table 5 shows a summary of the studies assessing the effects of Na intake on sweat electrolyte concentration and total sweat electrolyte loss during exercise and/or heat stress. The disparate results among studies may be reconciled in part by considering the time course of sweat glands’ response to variations in salt balance and associated changes in circulating aldosterone. As noted by Robinson in the early 1950s, while the renal system responds to a salt deficiency or excess within 1–3 h, the sweat glands typically require 1–4 days [134,238]. The literature summary in Table 5 is in general agreement with this notion. Indeed, most studies have shown that several days to weeks of dietary Na manipulation are associated with changes in sweat [Na] [45,224,225,235,236,239–241]. Other studies, usually of shorter duration (up to 3 days) [195,242] or with relatively small changes in daily Na ingestion [243,244] have reported no or minimal effect of dietary Na on sweat [Na] or the rate of Na excretion. The relation between acute (i.e. shortly before/during exercise) Na intake and sweat [Na] has not been well-studied. However, in one investigation, Hamouti et al. (2012) found no differences in sweat [Na] when various amounts of Na (0, 1.45 g, or 2.9 g) were ingested 1.5 h before exercise [245]. This result is perhaps not surprising based on the time course of sweat gland responsiveness, which is also in agreement with the notion that genomic effects of aldosterone on sweat [Na] are stronger than non-genomic actions (as discussed above in the Overview of Sweat Composition section) [150]. Regardless of duration, all studies have been consistent in finding no effect of salt deficiency or excess on sweating rate (Table 5). Therefore, any change in the rate of sweat NaCl excretion associated with dietary NaCl is likely due to changes in sweat concentrations.
Finally, it is important to discuss the dietary Na vs. sweat [Na] literature within a practical context. Several studies have employed study designs with large, perhaps unrealistic changes in dietary Na intake. For example, in five out of the 12 studies in Table 5 the high Na diet consisted of ≥8 g Na/day sustained over 5 days or more [45,224,240–242]. The low Na diet in these same studies was 0.5 to 2.3 g/day, resulting in vast differences in controlled daily Na intakes (by at least ~6 g). Few studies included “normal” dietary Na trials, which is 3.4 g/day for Americans [246]. The variation in sweat [Na] as a result of smaller deviations in Na intake, more realistic to a free-living individual, is yet to be fully elucidated. Nonetheless, in these five studies, the change in mean sweat [Na] was inconsistent, ranging from −5 mmol/L to +30 mmol/L. In addition, some studies measured sweat [Na] via regional techniques [240,243], which may not be indicative of changes at the whole-body level. Others have used a parallel study design where sweat [Na] was not matched between groups at baseline [241]. Thus, it is important that future studies address these and other methodological limitations as also pointed out by McCubbin and Costa [247].
Trace minerals
Several studies have investigated the hypothesis that dietary intake of trace minerals and vitamins influences sweat composition. However, most [230,231,248–251] but not all [184,185,252] studies reported no association between dietary intake of trace minerals (Zn, Fe, Ca, Cu) and their concentrations or excretion rates in sweat. This research has included acute supplementation (within ~24 h of sweat collection) as well as controlled and free-living chronic dietary intake of minerals and vitamins. Regardless of study duration, the impact of diet on sweat mineral and vitamin loss seems to be minimal, at least in healthy individuals with no known deficiencies. For example, Vellar et al. [251] measured whole-body cell-free sweat before and after giving an acute oral iron load (ferrous succinate tablets) to healthy men that led to nearly a twofold increase in serum [Fe]. There was no change in sweat [Fe] or sweating rate during 60 min of passive heat stress as a result of the acute iron load [251]. Similarly, Lug and Ellis [250] found no significant changes in sweat vitamin concentrations in healthy heat-acclimatized men after administration of a dietary supplement of 500 mg L-ascorbic acid during the 24 h before sweat collection. Furthermore, in a 30-day controlled diet study in healthy men, Jacob et al. [248] found no association between dietary intake of Zn, Cu, and Fe and whole-body sweat [Zn], [Cu], and [Fe], respectively.
A few studies have found a significant change in sweat mineral concentrations associated with dietary intake [184,185,252] and the commonality of these studies is that they included patient populations with known mineral deficiencies or involved controlled interventions designed to deplete and subsequently replete mineral stores of healthy subjects. For example, Milne et al. [252] measured daily whole-body sweat Zn loss during controlled periods of Zn intake. For the first 5 weeks, Zn intake was 8.3 mg/d, then reduced to 3.6 mg/d for the next 16 weeks, followed by an increase to 33.7 mg/d for the final 4 weeks of the study. Corresponding sweat Zn loss was 0.49 mg/d, 0.24 mg/d, and 0.62 mg/d, respectively; equivalent to a 51% decrease with Zn restriction and a 27% increase with excess dietary Zn [252]. It is also important to interpret these results within the context of the source of mineral concentrations found in sweat. As pointed out by Milne et al., [252] sweat samples included desquamated cell debris as well as cell-free eccrine sweat. Therefore, in this study [252] it is difficult to discern how much of the sweat Zn originated from the body surface (epidermal cells) versus the interstitial fluid (secreted by the eccrine sweat gland), as changes in body mineral homeostasis can impact the mineral stores of the skin as well as that of the interstitial fluid [184,185,253].
Some studies have compared mineral concentrations of cell-free and cell-rich sweat in Fe and Zn-deficient patient populations versus healthy normal controls [184,185]. Prasad et al. [184] found that [Fe] and [Zn] in cell-rich sweat was lower in patients versus the healthy control group. However, in cell-free sweat, only [Zn] was lower in patients, while there were no differences in [Fe] between Fe and Zn-deficient patients and healthy controls. This study suggests that most of the Fe collected at the skin surface originates from desquamated epithelial cells, while most of the Zn is present in the cell-free portion of sweat. This may also partly explain why an acute increase in blood [Fe] in the study by Vellar et al. [251] resulted in no change in cell-free sweat [Fe].
There are no known reabsorption or secretion mechanisms by which the eccrine sweat gland could actively conserve or preferentially excrete minerals. Therefore, any significant changes in sweat mineral concentrations would be expected to be a result of significant changes in the mineral content of interstitial fluid (impacting cell-free sweat) and/or epidermal cells (impacting cell-rich sweat). Therefore, sweat mineral concentrations may be altered in situations of depletion in intervention studies or chronic deficiencies in patient populations. Note that this is not necessarily evidence of a homeostatic mechanism; rather a result of passive transport of minerals in accordance with concentration gradients during secretion of primary sweat in the secretory coil (cell-free sweat) and an artifact of surface contamination (cell-rich sweat). Furthermore, the impact of diet on cell-rich and cell-free sweat mineral concentrations will differ depending upon the mineral of interest. As discussed above, Fe and Ca are found in much higher concentrations, and Zn in lower concentrations in cell-rich versus cell-free sweat [134,184,233,234,254]; further complicating the interpretation of study results. Future studies on diet, mineral balance, and sweat mineral losses should carefully choose the methodology employed and consider the source of the minerals measured in the sweat. Regardless, based on the available evidence to date, the take-home message for healthy individuals is that small fluctuations in dietary mineral intake (that do not significantly alter mineral status or whole-body stores) seem to have minimal impact on sweat mineral loss.
Sweating-induced deficiencies
Sodium chloride
Of all the substances lost in sweat, Na and Cl are lost in the highest concentrations. Therefore, it has been suggested that Na and Cl are the principal electrolytes whose loss may affect homeostasis [7,134,191]. Plasma [Na] is normally between 135 and 145 mmol/L and is a function of the mass balance of Na, K, and water [255,256]. Hyponatremia is defined as a plasma [Na] less than 135 mmol/L [257] and can be life-threatening depending upon the severity of plasma sodium dilution (e.g. <125–130 mmol/L) and the rapidity in which it occurs [256]. This is because a reduction in solute concentration in plasma promotes movement of water from the extracellular to intracellular space, which can cause swelling in the brain and/or congestion in the lungs [257]. Hyponatremia has been reported in healthy athletes [256], laborers [174,258,259], and soldiers [176,260], as well as clinical populations (e.g. psychogenic polydipsia) [261]. Based on mathematical models using the prediction equation developed by Ngyuen and Kurtz [255], plasma [Na] is most sensitive to changes in total body water and thus the primary cause of hyponatremia is an increase in body mass due to overdrinking (of water or other hypotonic fluid) relative to body water losses [256]. However, the model also predicts that plasma [Na] is moderately sensitive to changes in the mass balance of Na and K [262], such as through loss of electrolytes in sweat. Excessive sweat Na losses can exacerbate decreases in plasma [Na] caused primarily by overdrinking for a long period of time [263] (e.g. during a > 4 h endurance event). Hyponatremia has also been documented concomitant with dehydration, suggesting that in these cases excessive sweat Na loss was the primary etiology underlying a fall in plasma [Na] [174,259,264–266]. However, hypovolemic hyponatremia is rare compared with hypervolemic hyponatremia and usually requires excessive sweating over longer durations (>8 h) [264].
An individual’s sweat [Na] impacts their risk for developing hyponatremia in situations of prolonged thermoregulatory sweating. For example, according to Na balance prediction models [262], a 70 kg athlete drinking 800 ml/h of water while running at 10 km/h in an air temperature of 28°C would reach hyponatremia (plasma [Na] <130) in ~6–8 h if their sweat [Na] was 75 mmol/L. If an athlete in the same set of conditions had a sweat [Na] of only 25 mmol/L, the model predicts that plasma [Na] would still be above 135 mmol/L after 12 h [262]. Empirical evidence in line with these prediction models can be found in the case study literature; which report numerous instances where the combination of excessive sweating (physical labor, hot/humid conditions) and high sweat [Na] and [Cl] have precipitated the development of hyponatremia and hypochloremia [174–176,259,263,265–267]. For example, a systematic review paper reported that subacute (≤14 days) presentation of electrolyte disturbances in 172 cystic fibrosis patients was often associated with heat exposure (61% of cases) and excessive sweating (26% of cases) [265]. Other electrolyte imbalances reported alongside hyponatremia in these case studies include hypokalemia, hypochloremia, and/or metabolic alkalosis [174,176,259,265].
It is important to define or contextualize high sweat [Na] and [Cl], sometimes referred to as “salty sweat”. The individuals with hyponatremia referenced in the case studies were reported to have sweat [Na] values >80 mmol/L and sweat [Cl] >70 mmol/L as measured on the forearm using the pilocarpine-stimulated sweat test. According to normative data, a forearm sweat [Na] of 80 mmol/L is approximately two standard deviations above average [156] (Figure 5). “Salty sweat” has been observed in both healthy individuals [151,263,266,268,269] and cystic fibrosis patients [174–176,259,263,267,270]. Regardless of the underlying cause of the high sweat [Na] and [Cl], case reports and theoretical models alike demonstrate that excessive electrolyte losses through sweating can contribute to the development of Na and Cl imbalances.
Trace minerals and vitamins
There have been some suggestions that athletes may require dietary supplementation of certain trace minerals due in part to excessive losses in sweat. The two trace minerals that have received the most attention in terms of sweat-induced deficiencies are Ca and Fe. For example, the most recent consensus statement from the International Olympic Committee mentions that excess losses in sweat, in combination with other factors, may lead to suboptimal Fe status in athletes and therefore may require dietary supplementation [271]. Other papers have suggested that sweat or dermal Ca losses in athletes may contribute to reduced bone mineral density through stimulation of parathyroid hormone during training [226,272,273]. However, the balance of the evidence suggests that sweat losses probably contribute minimally to whole-body trace mineral and vitamin deficiencies [134,148,250,253,274–279].
First, it is important to reiterate that many of the studies reporting substantial trace mineral and vitamin losses in sweat have used methods (e.g. arm bag or other regional techniques, scraping methods, minimal cleaning, inclusion of initial sweat at start of exercise) [226,280–284] that overestimate sweat vitamin and mineral concentrations, including [Ca] and [Fe], by up to 2-3 fold [134,228,233,234]. For example, 65 years ago Robinson and Robinson [134] recognized that a primary source of Ca and Fe found in sweat is associated with desquamated cell debris, which is characteristic of the arm bag technique. Regional measures of sweat trace minerals are also higher and more variable (e.g. inter-regional differences) than that of whole body [285,286], which makes it difficult to draw conclusions about the amount of sweat Ca and Fe losses and impact on homeostasis.
Studies have shown that during an acute bout of 1–2 h exercise serum ionized [Ca] decreases, resulting in subsequent elevation of parathyroid hormone and activation of bone reabsorption. The long-term concern with this is a reduction in athletes’ bone mineral density throughout the course of training. While the underlying mechanisms are yet to be elucidated, one hypothesis is that the exercise-induced increase in PTH is triggered by sweat Ca loss. However, only one study has reported an association between sweat Ca loss and any measure of Ca homeostasis or bone mineral density. Barry and Kohrt [273] found a significant inverse correlation between sweat Ca losses (measured during 2-h cycling) and baseline bone mineral density at the hip, femoral neck, and femoral shaft (r = −0.6 to −0.8) in competitive male cyclists. While the cyclists’ bone mineral density decreased over the course of one year of training, there was no correlation between sweat Ca loss and changes in bone mineral density [273]. Several other studies have reported no association between sweat Ca loss and measures of Ca homeostasis (bone mineral density, parathyroid hormone, C-terminal telopeptide of Type I collagen, or bone-specific alkaline phosphatase) in female cyclists [287], male cyclists [288,289], basketball players [226], or firefighters [277].
It is important to note that Ca supplementation (or infusion) can attenuate increases in PTH and activation of bone resorption during exercise [278,287,288]; however, the underlying mechanism is apparently unrelated to replacement of sweat Ca loss. In addition to the lack of evidence discussed above, the timing of changes in Ca homeostasis during exercise does not agree with the sweat Ca loss hypothesis. As pointed out by Kohrt et al. [278], because the decrease in serum ionized [Ca] occurs early in exercise (first 15 min) it is unlikely that the extent of Ca loss would be large enough to impact Ca homeostasis. Furthermore, while in extreme circumstances excess mineral loss cannot be ruled out as a contributing factor to suboptimal trace mineral status [290], for most athletes the main routes of loss are likely through other avenues such as urine or the gastrointestinal tract [249,291,292]. Taken together, micronutrient supplementation does not seem to be necessary on the basis of sweat excretion during physical activity, provided that dietary intakes are normal [250].
Comparison of sweat gland and kidney function
Water conservation and excretion
The sweat glands are often compared to the nephrons of the kidneys, whose main function, among others, is to conserve the vital constituents of the body [293]. Indeed, sweat glands share some similarities with the renal system; as eccrine glands have mechanisms to conserve Na, Cl, and bicarbonate losses in sweat as discussed in detail in the Mechanisms of secretion and reabsorption section above. For example, in response to aldosterone, sweat glands increase Na reabsorption in the duct leading to a decrease in sweat [Na], albeit with a greater time lag than that of the kidneys. A vital function of the kidneys is to regulate body water balance, stimulating diuresis with overhydration and antidiuresis with hypohydration and/or heat stress. These adjustments are mediated through changes in renal water reabsorption in response to arginine vasopressin (AVP) concentrations in the plasma [294]. With hyperosmotic hypovolemia, AVP binds to vasopressin type 2 receptors of the distal tubule and collecting duct of the kidneys, stimulating aquaporin transport of water. It has been suggested that AVP might facilitate eccrine gland water reabsorption in a similar manner, resulting in attenuated sweating rates and more concentrated sweat (as a consequence of water removal from the primary fluid along the duct) [295–298]. However, the majority of studies have concluded that neither administration of AVP (e.g. subcutaneous injection of pitressin) nor suppression of its effects (e.g. via ethanol ingestion) alter sweating rate or sweat electrolyte concentrations during heat exposure or exercise [193,299–305]. These studies also reported no correlation between plasma AVP concentrations and sweating rate or sweat [Na] [151,302,306]. Moreover, one study showed that pharmacological manipulation of vasopressin type 2 receptors with an agonist (desmopressin) or antagonist (tolvaptan) prior to exercise had no effect on sweat [Na] [306]. These results may be explained in part by the relatively sparse ductal membrane expression of aquaporin-5 compared with the secretory coil [151]. Taken together it appears that AVP does not regulate water loss via the sweat glands as it does in the kidneys; and the sweat duct does not play an important role in water conservation during exercise-heat stress [210,301,306,307]. Additionally, a recent study suggests that intradermal administration of atrial natriuretic peptide, a cardiac hormone that promotes urinary excretion of sodium and water, has no effect on sweating rate in young adults nor does it affect sweating in response to muscarinic receptor activation [308].
Excretion of toxicants
The notion that sweating is a means to accelerate the elimination of persistent environmental contaminants from the human body has been around for many years [309,310]. Detoxification methods include several hours per day of sauna bathing to stimulate excessive sweating, resulting supposedly in purification of the body and release of toxins from the blood. Some proponents of this method claim that increasing sweating via exercise or heat stress (sauna) is an effective clinical tool to protect against or overcome illness and disease [311–313]. Others suggest that physical activity leads to better health outcomes as a result of accelerated toxin elimination via thermal sweating [314,315]. As attractive as this idea sounds, there is little if any evidence to date that supports these claims [309,310].
In a series of studies, Genuis et al. [311–313,316–318] measured the concentration of environmental toxicants in the blood, sweat, and urine of humans. The overall finding of these studies was that many chemicals, including persistent organic pollutants, heavy metals, bisphenol A (BPA), and phthalate are excreted in sweat. Interestingly, the concentrations in sweat were often higher than that of blood and/or urine, and in some cases, chemicals were detected in sweat but absent in blood and urine. Such reports [280,314,318] have led some to hypothesize that these chemicals are perhaps preferentially excreted in sweat to reduce the body burden. However there are several important methodological limitations to consider when measuring environmental toxicants in sweat. First, many of these studies used sweat collection methods that are susceptible to surface contamination and sweat evaporation, which would artificially increase the concentration of toxicants measured in sweat samples. For instance, in most of these studies [311–314,316–318], sweat was collected by the subjects on their own (uncontrolled, unsupervised), from any site on their body, by scraping sweat from the skin surface with a stainless steel spatula into a glass jar. With these methods, it is probable that sweat samples were tainted with sebum secretions. Scraping methods increase the likelihood of skin surface (epidermal cells) contamination because scraped sweat contains 4-10x more lipid than clean sweat [319]; potentially explaining the high concentrations of some the of lipophilic toxicants in sweat. Furthermore, the method of sweat stimulation (exercise, sauna) and timing (with respect to how long sweating had commenced before collection) were not controlled [311–314,316–318]. Other studies [280,320] used the arm bag method which is also susceptible to skin surface contamination. As previously discussed the epidermis contains many contaminants, including heavy metals measured in these studies (arsenic and lead) [321,322].
When using these methods Genuis et al. [311] found consistently higher concentrations of BPA in sweat than urine. Furthermore, BPA was detected in the sweat of 16 of the 20 subjects, but only two of the 20 subjects had BPA in their serum. In another study, PCB52 concentration was higher in sweat than blood and urine [316]. Given that interstitial fluid is the precursor to primary sweat secretion it is unlikely that the BPA or PCB52 collected at the skin surface in these studies can be attributed to eccrine sweat if the chemical is absent in the blood. Instead the chemicals could have originated from sebum secretions or epidermal cell contamination. One study lends support for this line of thinking: Porucznik et al. [323] collected sweat (via PharmChek absorbent patches) and urine for 7 days and found BPA in urine, but an absence of BPA in sweat. For example, the highest measured urine BPA concentration was 195 ng/ml for an individual with deliberate exposure, but no BPA was detected above background in the corresponding sweat patch. These results suggest that the renal system is primarily responsible for BPA elimination from the body and pharmacokinetic studies provide additional support for this: in humans, it has been shown that 84–97% of BPA is eliminated in urine 5–7 h after exposure and 100% is eliminated after 24 h [324]. The primary avenue for heavy metals excretion, based on tracer studies, is fecal output [325]. Meanwhile, there are no known mechanisms by which the sweat glands would preferentially secrete (concentrate) BPA, persistent organic pollutants, and trace metals to facilitate transport out of the body. Thus direct evidence for sweating as an effective detoxification method is lacking.
Still, future well-controlled studies designed to collect clean eccrine sweat are needed to clarify or refute any potential role of sweating as a therapeutic tool to eliminate toxins from the body. While therapeutic health benefits (mostly subjective measures) from detoxification protocols in some patient populations have been documented, it is important to note that sauna is only one component of a holistic intervention [310]. Most protocols also include several weeks of strict changes in diet, exercise, and sleep and therefore it is not possible to attribute any benefit solely to sauna therapy [309,310,326–328]. Moreover, the sauna protocols used in these studies have employed 2–5 h/day of excessive sweating. The efficacy of lower rates of sweat loss, more realistic to the context of everyday life, is unknown [309].
Excretion of ethanol
Another perceived function of the eccrine sweat glands is the elimination of ethanol from the body through increases in sweating rate and/or sweat ethanol concentration. In fact, increased sweating is often considered a hangover symptom and is part of the Alcohol Hangover Severity Scale used as the standard in alcohol hangover research [329]. Furthermore, it is commonly believed that an effective cure for hangovers after heavy drinking is to stimulate sweating (via exercise or sauna bathing) to accelerate recovery from alcohol intoxication. However, the evidence to date does not support these ideas; not to mention there are significant health concerns with sauna bathing during alcohol hangover [330].
In a validation study, the 12-symptom Alcohol Hangover Severity Scale questionnaire (which includes perceived sweating as well as fatigue, dizziness, clumsiness, thirst, nausea, and others) had a hangover severity predictive validity of r2 = 0.92 [329]. Interestingly though, perceived sweating was not significantly different between the hangover and control groups in this naturalistic study, while all other individual symptoms successfully differentiated between the two conditions [329]. Furthermore, alcohol intake has been found to have no or minimal impact on sweating rate in laboratory intervention studies [302,331–333]. For instance, two separate studies found no differences in regional sweating rate (chest or upper arm) in response to hot water immersion [332] or exercise-heat stress [331] after alcohol ingestion (that lead to 0.07–0.11 g/dl blood alcohol concentration) compared with the placebo conditions. One study did find a higher chest sweating rate during passive heat stress (33°C) 10–30 min after 0.36 g/kg alcohol ingestion compared with water. However, the elevated sudomotor response was transient, as sweating rate decreased after 30 min and became even with the water trial by 40 min into heating [333]. In addition, differences in sweating rates were very low (up to 0.1–0.2 mg/cm2/min) and unlikely to be of practical significance from a detoxification perspective. On the whole-body level, the difference would be equivalent to ≤100 g over the course of 30 min in a 1.8 m2 individual, but this is likely to be an overestimate since chest sweating rate is higher than that of whole body. On this point, no study to the author’s knowledge has measured changes in whole-body sweating rate in response to alcohol ingestion, either immediately after ingestion or during alcohol hangover.
It does seem that sweat ethanol concentration increases with ethanol ingestion and rises linearly with increases in blood alcohol concentration. For example, Buono et al. collected serial sweat samples (using the anaerobic technique) via pilocarpine iontophoresis for 3 h after 13 mmol/kg ethanol ingestion and found a significant correlation between blood versus sweat ethanol concentrations and the slope of the relation was 1.01 [334,335]. This nearly identical ethanol concentration between blood and sweat supports the idea that sweat ethanol originates from the interstitial fluid and its concentration is not significantly altered during transport through the duct onto the skin surface; which is counter to the suggestion that the sweat glands have homeostatic mechanisms to detoxify the blood (via concentrating mechanisms). Moreover, the main avenue of ethanol elimination from the body is known to be via oxidation by alcohol dehydrogenase and aldehyde dehydrogenase eventually breaking ethanol down to acetyl CoA, all of which occurs in the liver. It is in this manner that 90% of alcohol is removed from the body, with the other 10% being excreted in breath, sweat, and urine [336]. Taken together the available evidence suggests that sweating likely plays a very small role in alcohol detoxification or hangover cures.
Excretion of metabolic waste
Another important function of the kidneys is excretion of metabolic and dietary waste products. Since some waste products appear in sweat the eccrine glands are also thought of as an excretory organ. For example, sweat contains urea, the major nitrogen-containing metabolic product of protein catabolism. According to Sato [15], urea readily crosses the eccrine glandular wall and cell membrane and therefore concentrations of urea in sweat are expected to be about the same as that of the plasma. Some studies report very high urea concentrations in sweat [337–339], up to 50x that of serum [337], and suggest that this is evidence for a selective transport mechanism across the sweat gland, especially in patients with kidney damage, to clear the blood of high urea concentrations [337]. However, many of these studies used methods susceptible to sample evaporation (collection of sweat drippage) [337,339] or surface contamination (sweat collected at onset of exercise) [338], which can lead to artificial increases in sweat urea concentrations (see Table 2). Other studies have shown that uric acid and creatinine excretion via sweat is insignificant compared with elimination rates through the kidneys [337,339]. Taken together, there is limited evidence that the sweat glands excretory function makes a substantial contribution to homeostasis [134,193].
Altered sweat gland function from conditions and medications
As shown in Table 6, certain medical conditions and medications can impact sweating rate and sweat composition. As discussed in the Thermoregulation section above, evaporation of sweat is crucial for temperature regulation in warm conditions and this is evident in patients suffering from anhidroses. Diabetes mellitus, multiple sclerosis, spinal cord injury, and anhidrotic ectodermal dysplasia are associated with a significant reduction in sweating; which, in some cases, can severely impair one’s ability to dissipate heat during higher thermal loads [340–344]. In particular, heat intolerance is well documented in patients with anhidrotic ectodermal dysplasia, a genetic condition resulting in a paucity of sweat glands over the entire body surface [3,15]. In addition, the important function of salt conservation by the sweat gland is evident in patients with reduced ion reabsorptive capacities due to a genetic deficiency or absence of functioning CFTRs (cystic fibrosis) or impaired adrenal cortex function (Addison’s disease) [345,346]; who may be more susceptible to electrolyte imbalances [174–176,259,263,265,267].
Table 6.
Conditions and medications that alter sweat gland function.
TimingEffect on sweating rate and/or sweat composition
Selected conditions and medications | ||
Cystic fibrosis | Chronic | Higher sweat [Na] and [Cl] than normal because of a genetic deficiency or absence of functioning CFTR leading to lower Na and Cl reabsorption rates in the sweat duct [167,168,170] |
Addison’s disease | Chronic | Higher sweat [Na] and [Cl] than normal because of impaired adrenal cortex function (aldosterone secretion) leading to lower Na and Cl reabsorption rates in the sweat duct [345,346] |
Diabetes mellitus | Chronic | Reduced sweating with T1DM and T2DM; potential mechanisms related to autonomic neuropathy and reduced thermosensitivity, reduced maximal sweating rate, and/or lower number of active sweat glands; impaired ability to dissipate heat, especially during higher thermal loads and in individuals with lower fitness level [340–344] |
Multiple sclerosis | Chronic | Reduced sweating because of lesions within central nervous system leading to reduced sweat output per gland [459] |
Spinal cord injury | Chronic | Reduced or complete absence of sweating in the insensate skin due to disruption in neural pathways involved in central and peripheral control of sweating [460,461]; compensatory increase in sweating occurs in sensate skin above the spinal injury [462,463] |
Severe burns and skin grafting | Chronic | Reduced or complete absence of sweating in the burned area because entire epidermal and majority of the dermal layer (including sweat glands) are excised. Disruption in sweating remains even as the skin graft heals [347–349] |
Sunburn | Acute | Reduced sweating in artificially-induced mildly sunburned skin [350] |
Miliaria rubra (heat rash or prickly heat) | Acute | Reduced sweating because of pore occlusion via keratin plugs causing mechanical blockage of sweat flow onto skin surface; caused by high humidity (excessive sweat) on skin surface for long duration [351,352] |
Atopic dermatitis (eczema) | Episodic | Reduced sweating onto the skin surface because of obstruction of sweat pores by keratin plugs, leakage of sweat into dermal tissue around the glands, and/or potentially histamine-induced sweat suppression [215,353,354]; sweat glucose concentration may be higher than normal with acute atopic dermatitis [464] |
Anhidrotic ectodermal dysplasia | Chronic | Reduced or complete lack of sweating because of genetic paucity or absence of sweat glands over entire body surface [3] |
Primary hyperhidrosis | Chronic/Episodic | Increased sweating with focal or bilateral distribution affecting primarily the axilla, palms, soles, and craniofacial areas [356,359]. Etiology involves neurogenic overactivity of otherwise normal sweat glands [3,29]; associated with genetic predisposition [359,465]. Limited data on sweat composition. |
Secondary hyperhidrosis | Chronic/Episodic | Increased sweating with generalized or unilateral distribution as a result of underlying physiologic condition (fever, pregnancy, menopause), pathology (malignancy, infection, cardiovascular disease, endocrine/metabolic, neurological or psychiatric disorders), or medication [3,356–359]. Limited data on sweat composition. |
Tattoos | Chronic | Reduced sweating rate and higher sweat [Na] in response to pharmacologically-induced local sweating than non-tattooed skin; unknown etiology [466–468]. More research involving exercise or heat-induced whole body sweating is needed. |
Medications | Acute/ Chronic | Antimuscarinic anticholinergic agents, carbonic anhydrase inhibitors, and tricyclic antidepressants can cause generalized hypohydrosis; cholinesterase inhibitors, SSRI, opioids, and TCA can cause generalized hyperhidrosis [346,355]. Limited data on sweat composition. |
CFTR: cystic fibrosis transmembrane conductance regulator; SSRI: selective serotonin reuptake inhibitors; T1DM and T2DM, type 1 and 2 diabetes mellitus; TCA: tricyclic antidepressants
Other conditions associated with reduced sweating include burns and skin grafting [347–349], sunburn [350], miliaria rubra [351,352], and atopic dermatitis [215,353,354], as well as medications that interfere with neural sudomotor mechanisms (e.g. anticholinergics and tricyclic antidepressants) [346,355]. Hyperhidrosis, where sweating occurs in excess of thermoregulatory demands, can occur with primary etiology [3,29] or secondary to physiologic condition (fever, pregnancy, menopause), pathology (malignancy, endocrine, metabolic, or psychiatric disorder), or medication (cholinesterase inhibitors, SSRIs, opioids) [3,356–359]. However, these types of hypo- and hyperhidrosis are often localized and/or episodic and the impact on whole-body thermoregulation and/or fluid balance during exercise and/or heat stress is not well-studied. The reader is referred to the supporting references in Table 6 for more details on each of the conditions and medications that alter sweat gland function.
Conclusions
This paper discussed sweat gland physiology and the state of the evidence regarding various roles of sweating and sweat composition in human health. Based on this review of the literature, the following conclusions were drawn:
Biography
•
Lindsay B. Baker, PhD, is Senior Principal Scientist at the Gatorade Sports Science Institute (GSSI) and a PepsiCo R&D Associate Fellow. Lindsay has been conducting sports nutrition, hydration, and sweat studies for the GSSI research program since 2007. She is a Fellow of the American College of Sports Medicine and is on the Scientific Advisory Board for the Korey Stringer Institute.
Funding Statement
This work was supported by the Gatorade Sports Science Institute, a division of PepsiCo, Inc.
Abbreviations
ASGDactivated sweat gland densityAVParginine vasopressinATPadenosine triphosphateBPAbisphenol-ACacalciumCFTRcystic fibrosis transmembrane conductance regulatorClchlorideCucopperEreqevaporative requirement for heat balanceFeironHCO3bicarbonateKpotassiumMgmagnesiumMnmangeneseNasodiumNH3ammoniaRSRregional sweating rateSGOsweat gland outputWBSRwhole body sweating rateZnzinc
Disclosure statement
The author is employed by the Gatorade Sports Science Institute, a division of PepsiCo, Inc. The views expressed in this article are those of the authors and do not necessarily reflect the position or policy of PepsiCo, Inc.
References
[1] Casa DJ, Cheuvront SN, Galloway SD, et al. Fluid needs for training, competition, and recovery in track-and-field athletes. Int J Sport Nutr Exerc Metab. 2019;29(2):175–180. [PubMed] [Google Scholar]
[2] Shibasaki M, Davis SL.. Human perspiration and cutaneous circulation In: Meyer F, Szygula Z, Wilk B, editors. Fluid balance, hydration, and athletic performance. Boca Raton (FL): CRC Press; 2016. p. 33–58. [Google Scholar]