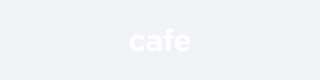
Guidelines for measuring reactive oxygen species and oxidative damage in cells and in vivo
- Consensus Statement
- Published: 27 June 2022
Guidelines for measuring reactive oxygen species and oxidative damage in cells and in vivo
- Michael P. Murphy,
- Hülya Bayir,
- Vsevolod Belousov,
- Christopher J. Chang,
- Kelvin J. A. Davies,
- Michael J. Davies,
- Tobias P. Dick,
- Toren Finkel,
- Henry J. Forman,
- Yvonne Janssen-Heininger,
- David Gems,
- Valerian E. Kagan,
- Balaraman Kalyanaraman,
- Nils-Göran Larsson,
- Ginger L. Milne,
- Thomas Nyström,
- Henrik E. Poulsen,
- Rafael Radi,
- Holly Van Remmen,
- Paul T. Schumacker,
- Paul J. Thornalley,
- Shinya Toyokuni,
- Christine C. Winterbourn,
- Huiyong Yin &
- Barry Halliwell
Nature Metabolism volume 4, pages651–662 (2022)Cite this article
Abstract
Multiple roles of reactive oxygen species (ROS) and their consequences for health and disease are emerging throughout biological sciences. This development has led researchers unfamiliar with the complexities of ROS and their reactions to employ commercial kits and probes to measure ROS and oxidative damage inappropriately, treating ROS (a generic abbreviation) as if it were a discrete molecular entity. Unfortunately, the application and interpretation of these measurements are fraught with challenges and limitations. This can lead to misleading claims entering the literature and impeding progress, despite a well-established body of knowledge on how best to assess individual ROS, their reactions, role as signalling molecules and the oxidative damage that they can cause. In this consensus statement we illuminate problems that can arise with many commonly used approaches for measurement of ROS and oxidative damage, and propose guidelines for best practice. We hope that these strategies will be useful to those who find their research requiring assessment of ROS, oxidative damage and redox signalling in cells and in vivo.
요약
활성산소종(ROS)의 다양한 역할과 건강과 질병에 미치는 영향이
생물 과학 전반에 걸쳐 밝혀지고 있습니다.
이러한 발전으로 인해
ROS의 복잡성과 그 반응에 익숙하지 않은 연구자들은
상용 키트와 프로브를 사용하여
ROS와 산화적 손상을 부적절하게 측정하고,
ROS(일반적인 약어)를 마치 개별 분자 실체처럼 취급하게 되었습니다.
안타깝게도 이러한 측정값을
적용하고 해석하는 데는
많은 어려움과 한계가 있습니다.
이로 인해 개별 ROS와 그 반응,
신호 분자로서의 역할,
산화적 손상을 가장 잘 평가하는 방법에 대한 지식이 확립되어 있음에도 불구하고
오해의 소지가 있는 주장이 문헌에 등장하여
발전을 저해하는 결과를 초래할 수 있습니다.
이 합의문에서는
ROS 및 산화적 손상을 측정하는 데
일반적으로 사용되는 여러 접근 방식에서 발생할 수 있는 문제를 조명하고
모범 사례에 대한 가이드라인을 제안합니다.
이러한 전략이
세포와 생체 내에서 ROS,
산화적 손상 및 산화 환원 신호의 평가가 필요한 연구를 하는 분들에게
도움이 되기를 바랍니다.
Similar content being viewed by others
Defining roles of specific reactive oxygen species (ROS) in cell biology and physiology
Article 21 February 2022
Reactive oxygen species (ROS) as pleiotropic physiological signalling agents
Article 30 March 2020
Fundamentals of redox regulation in biology
Article 30 April 2024
Main
Reactive oxygen species (ROS) (Box 1) are intimately involved in redox signalling but in some situations can also lead to oxidative damage. Hence, they have both physiological and pathophysiological roles in biology1,2,3,4. Consequently, researchers from diverse fields often need to measure ROS, to assess oxidative events and to investigate their biological importance using antioxidants (Box 1) or inhibitors to modulate the phenomena observed. There are many assays and commercial kits available, but their use and interpretation are challenging and open to artefacts. There is a well-established field of biophysics/biochemistry/chemistry focusing on the identification of ROS, their chemical reactions and products of oxidative damage. However, as with many specialized fields, this literature can be hard to interpret by those working outside the area. Frequently problems arise due to reliance on commercial kits that claim to measure ‘ROS’ or ‘oxidative damage’, or from the use of ‘antioxidants’ in general terms, when progress requires understanding of specific molecular mechanisms.
To address these points, this international group has set out guidelines on the nomenclature and measurement of ROS, oxidative reactions and oxidative damage. Our focus is on the techniques used to measure ROS and oxidative damage. These can be applied to their role in pathology, but it is also important to note that changes in the levels of ROS and consequent changes in the activity of redox-sensitive cellular processes are central to the field of redox signalling1,2,3,4. We hope that these guidelines will be useful for researchers who find themselves carrying out experiments in this area. These topics, and indeed the approaches we advocate, have been covered by many reviews in the past1,2,3,4,5,6,7,8,9,10,11 and which researchers are strongly encouraged to read. Here we distill the key points underlying this consensus statement.
주요
활성산소(ROS)(상자 1)는
산화 환원 신호에 밀접하게 관여하지만
일부 상황에서는 산화적 손상을 일으킬 수도 있습니다.
따라서
활성산소는
생물학에서 생리적 및 병리 생리학적 역할을 모두 수행합니다1,2,3,4.
따라서
다양한 분야의 연구자들은 산화 현상을 평가하고
항산화제(상자 1) 또는
억제제를 사용하여 관찰된 현상을 조절하기 위해
ROS를 측정하고 생물학적 중요성을 조사해야 하는 경우가 많습니다.
많은 분석법과 상용 키트가 시중에 나와 있지만,
그 사용과 해석이 까다롭고
오류에 노출되어 있습니다.
생물물리학/생화학/화학 분야에서는
ROS와 그 화학 반응 및 산화적 손상의 생성물을 식별하는 데
중점을 두는 잘 정립된 분야가 있습니다.
그러나
많은 전문 분야와 마찬가지로
이 분야 외부에서 일하는 사람들은
이 문헌을 해석하기 어려울 수 있습니다.
'ROS' 또는 '산화적 손상'을 측정한다고 주장하는
상업용 키트에 의존하거나
특정 분자 메커니즘에 대한 이해가 필요한 경우
일반적인 용어로 '항산화제'를 사용함으로써 문제가 발생하는 경우가 종종 있습니다.
이러한 점을 해결하기 위해
이 국제 그룹은
ROS,
산화 반응 및 산화 손상의 명명법과
측정에 관한 가이드라인을 마련했습니다.
저희는
ROS와 산화적 손상을 측정하는 데 사용되는
기술에 초점을 맞추고 있습니다.
이는
병리학에서의 역할에 적용될 수 있지만,
ROS 수준의 변화와 그에 따른
산화 환원 민감성 세포 과정의 활성 변화는
산화 환원 신호1,2,3,4 분야의 핵심이라는 점에 주목하는 것도 중요합니다.
이 가이드라인이
이 분야의 실험을 수행하는
연구자들에게 도움이 되기를 바랍니다.
이러한 주제와 실제로 우리가 지지하는 접근 방식은 과거에 많은 리뷰1,2,3,4,5,6,7,8,9,10,11에서 다루어졌으며, 연구자들은 이를 읽어보시기를 강력히 권장합니다. 여기에서는 이 합의 성명서의 근간이 되는 핵심 사항을 요약합니다.
Box 1 Definitions
Reactive oxygen species (ROS) is a collective term for species derived from O2 that are more reactive than O2 itself. The term includes not only the superoxide radical anion (O2•−) and some other oxygen radicals, but also some non-radical derivatives of O2 such as hydrogen peroxide (H2O2), hypochlorous acid (HOCl) and peroxynitrite/peroxynitrous acid (ONOO−/ONOOH). Hence all oxygen radicals are ROS, but not all ROS are radical species (the latter being defined as a species with one or more unpaired electrons). ‘Reactive’ is a relative term; O2•− and H2O2 are selective in their reactions with biological molecules, leaving most of them unscathed, whereas •OH attacks everything (Table 1).
활성산소종(ROS)은
산소 자체보다 반응성이 강한 산소(O2)에서 파생된 종을
통칭하는 용어입니다.
이 용어에는
슈퍼옥사이드 라디칼 음이온(O2--) 및
기타 산소 라디칼뿐만 아니라
과산화수소(H2O2),
차아염소산(HOCl),
과산화아질산염/과산화질산(ONOO-/ONOOH) 등
O2의 일부 비라디칼 유도체도 포함됩니다.
https://www.ncbi.nlm.nih.gov/pmc/articles/PMC8045585/
https://www.ncbi.nlm.nih.gov/pmc/articles/PMC4712174/
따라서
모든 산소 라디칼은
ROS이지만
모든 ROS가 라디칼 종은 아닙니다(후자는 하나 이상의 짝을 이루지 않은 전자를 가진 종으로 정의됨).
'반응성'은
상대적인 용어입니다.
O2-- 및 H2O2는
생물학적 분자와의 반응에서 선택적으로 반응하여
대부분의 분자를 손상시키지 않는 반면
-OH는 모든 분자를 공격합니다(표 1).
Superoxide radical anion | O2•− | Selectively reactive, does not attack most biological molecules Can reduce transition metals (Fe3+, Cu2+), reaction rate depends on the metal ion ligand Reacts very rapidly with nitric oxide (k2 > 109 M−1 s−1) to yield peroxynitrite: O2•− + NO• → ONOO− and with other radicals to form hydroperoxides: O2•− + R• + H+ → ROOH Can damage certain enzymes that contain Fe–S clusters |
Hydrogen peroxide | H2O2 | Unreactive with most biomolecules Reacts slowly with most thiols—for example, k ≈ 1 M−1 s−1 for GSH—but more rapidly with certain protein Cys residues, particularly those with low pKa Reacts with certain transition metal ions to give •OH (rate constants 102–107 M−1 s−1, depending on the metal and ligands to the metal ion) Main biological reactions are with haem, thiols and peroxidase enzymes Reacts with CO2 to form the more reactive peroxymonocarbonate (HCO4−)50 |
Hydroxyl radical | •OH | Indiscriminately reactive; reacts with whatever is adjacent to it at near diffusion-controlled rates |
Peroxynitrite (the physiological mixture of peroxynitrite, ONOO− and its more reactive protonated form, peroxynitrous acid, ONOOH; pKa 6.8) | ONOO−/ONOOH | Direct reactions with thiols and transition metal centres up to 107 M−1 s−1; reacts with CO2 to give nitrosoperoxycarbonate (ONOOCO2−) Peroxynitrite itself can oxidize several biomolecules or homolysis of peroxynitrous acid can generate •OH ONOOH → NO2• + •OH, although a more prominent reaction is ONOOH → NO3−+H+ ONOO− + CO2 → ONOOCO2− → minor (CO3•− + NO2•) + major (CO2+NO3−) |
Carbonate radical anion | CO3•− | Formed from reaction of CO2 with peroxynitrite (see above) and also from reaction of HCO3− with •OH. Fairly reactive, oxidizes guanine in DNA, cysteine, tyrosine and tryptophan |
Hypohalous acids (hypochlorous, hypobromous acids) | HOCl HOBr | Strong oxidants, major reactions are with thiols and methionine Reactions with amines generate secondary chloramines/bromamines, which retain less (but still considerable) oxidizing ability React rapidly with thiocyanate (SCN−), present at high levels in many body fluids, to generate HOSCN (which is also generated by peroxidases); HOSCN is less reactive and highly specific for thiols |
Singlet oxygen | 1O2 | Two singlet states of O2 exist, although only the 1Δg state (not a free radical) is of major biological relevance. The singlet electron configuration makes this state much more reactive than ground-state triplet O2. Can be formed by photosensitization reactions in which molecules such as porphyrins, riboflavin, bilirubin and chlorophyll absorb light and transfer this energy to ground-state O2, and also via chemical reactions of peroxyl radicals and HOCl, amongst others |
Nitrogen dioxide radical | NO2• | A major atmospheric pollutant. Also generated from peroxynitrite (see above) and oxidation of nitrite (NO2−) by peroxidase enzymes. Rapidly oxidizes electron-rich compounds (for example, ascorbate and thiols). Undergoes addition reactions with radicals derived from tyrosine, tryptophan, lipids and DNA bases (for example, guanine) to give nitrated products (for example, 3-nitrotyrosine, nitrotryptophans, nitrolipids and nitrated DNA bases). Some nitrated products have signalling functions |
과산화 라디칼 음이온
O2--
선택적 반응성, 대부분의 생물학적 분자를 공격하지 않음
전이 금속(Fe3+, Cu2+) 환원 가능, 반응 속도는 금속 이온 리간드에 따라 달라짐
산화 질소와 매우 빠르게 반응하여(k2>109M-1s-1) 퍼옥시나이트르라이트를 생성합니다:
O2-- + NO- → ONOO-
그리고 다른 라디칼과 반응하여 하이드로퍼옥사이드를 형성합니다:
O2-- + R- + H+ → ROH
Fe-S 클러스터를 포함하는 특정 효소를 손상시킬 수 있습니다.
과산화수소
H2O2
대부분의 생체 분자와 반응하지 않음
대부분의 티올(예: GSH의 경우 k≈ 1 M-1s-1 )과 느리게 반응하지만 특정 단백질 Cys 잔기, 특히 pKa가 낮은 잔기와는 더 빠르게 반응합니다.
특정 전이 금속 이온과 반응하여 -OH를생성합니다(금속 및 금속 이온에 대한 리간드에 따라 속도 상수 102-107M-1s-1).
주요 생물학적 반응은 헤모글로빈, 티올 및 퍼옥시다아제 효소와의 반응입니다.
CO2와 반응하여 반응성이 더 높은 과산화수소(HCO4-)50를 형성합니다.
하이드록실 라디칼
-OH
무차별적으로 반응하며, 거의 확산 제어된 속도로 인접한 모든 것과 반응합니다.
퍼옥시니트라이트 (생리적 혼합물인 퍼옥시니트라이트, ONOO-와 그보다 반응성이 높은 양성자화된 형태인 퍼옥시니트로스산, ONOOH; pKa 6.8)
ONOO-/ONOOH
티올 및 전이 금속 중심과 최대107M-1s-1의 직접 반응;CO2와 반응하여 니트로소퍼옥시카보네이트(ONOOCO2-)를 생성합니다.
과산화아질산염 자체는 여러 생체 분자를 산화시키거나 과산화아질산의 자가분해로 -OH를생성할 수 있습니다.
ONOOH → NO2-+-OH,
더 두드러진 반응은
ONOOH →
ONOO-+CO2 → ONOOCO2- → 마이너(CO3-- + NO2-)+ 메이저입니다.
탄산염 라디칼 음이온
CO3--
CO2와 퍼옥시니트라이트(위 참조)와의 반응 및 -OH와의반응으로 형성됩니다. 반응성이 매우 강하고 DNA의 구아닌, 시스테인, 티로신 및 트립토판을 산화시킵니다.
차아염소산 (차아 염소산, 차아 브롬산)
HOCl
HOBr
강력한 산화제, 주요 반응은 티올 및 메티오닌과의 반응입니다.
아민과의 반응은 이차 클로라민/브로마민을 생성하며, 이는 산화 능력이 덜하지만 여전히 상당한 양을 유지합니다.
많은 체액에 높은 수준으로 존재하는 티오시아네이트(SCN-)와 빠르게 반응하여 HOSCN(퍼옥시다아제에 의해서도 생성됨)을 생성합니다; HOSCN은 반응성이 낮고 티올에 대해 매우 특이적입니다.
단일 산소
1O2
O2의 두 가지 단일 상태는 존재하지만, 1Δg 상태(자유 라디칼이 아님)만이 생물학적으로 중요한 관련이 있습니다. 단일 전자 구성으로 인해 이 상태는 기저 상태 삼중수소 O2보다 훨씬 더 반응성이 높습니다. 포르피린, 리보플라빈, 빌리루빈, 엽록소와 같은 분자가 빛을 흡수하여 이 에너지를 지상 상태의 O2로 전달하는 광감작 반응과 퍼옥실 라디칼 및 HOCl 등의 화학 반응을 통해 형성될 수 있습니다.
이산화질소 라디칼
NO2-
주요 대기 오염 물질입니다. 아질산과산화효소에 의한 아질산염()의 산화(위 참조)에서도 생성됩니다. 전자가 풍부한 화합물(예: 아스코르브산염 및 티올)을 빠르게 산화시킵니다. 티로신, 트립토판, 지질 및 DNA 염기(예: 구아닌)에서 파생된 라디칼과 추가 반응을 일으켜 질산염 생성물(예: 3-니트로티로신, 니트로트립토판, 니트로지질 및 질산염 DNA 염기)을 생성합니다. 일부 질산염 제품에는 신호 기능이 있습니다.
Antioxidant is a term often used but difficult to define clearly.
When ROS are generated in vivo, many antioxidants come into play. Their relative importance depends upon:
항산화제는 자주 사용되지만 명확하게 정의하기 어려운 용어입니다.
생체 내에서 ROS가 생성될 때 많은 항산화제가 작용합니다. 항산화제의 상대적 중요성은
- 어떤 ROS가 어떤 양으로, 어떤 시간 경과에 걸쳐 생성되는가?
- 생성되는 방법과 장소
- ROS에 의한 손상의 목표가 측정되는 대상
One definition of an antioxidant is "any substance that delays, prevents or removes oxidative damage to a target molecule"1. There is no universal ‘best’ antioxidant: different antioxidants react with different ROS at variable rates, act in various locations and protect different molecular targets. An alternative definition is "a substance that reacts with an oxidant to regulate its reactions with other targets, thus influencing redox-dependent biological signalling pathways and/or oxidative damage".
Oxidative damage: the biomolecular damage caused by the attack of ROS upon the constituents of living organisms. Increased levels of oxidative damage can occur from increased ROS production but also from decreased repair or removal processes—for example, failure to clear oxidized proteins or repair oxidized DNA sufficiently rapidly: both can happen in certain diseases.
Biomarker: can be defined as any substance, structure or process that can be measured in the body or its products and influence or predict the incidence of outcome or disease54.
항산화제의 한 가지 정의는
"표적 분자에 대한 산화적 손상을 지연, 예방 또는 제거하는 물질"1입니다.
항산화제는
다양한 ROS와 다양한 속도로 반응하고
다양한 위치에서 작용하며
다양한 분자 표적을 보호하기 때문에 보편적인
'최고의' 항산화제는 존재하지 않습니다.
다른 정의로는
"산화제와 반응하여
다른 표적과의 반응을 조절하여
산화 환원 의존적 생물학적 신호 경로 및/또는
산화적 손상에 영향을 미치는 물질"을 들 수 있습니다.
산화적 손상: 생체 구성 요소에 대한 ROS의 공격으로 인해 발생하는 생체 분자 손상.
산화적 손상의 증가는 ROS 생성 증가로 인해 발생할 수 있지만, 복구 또는 제거 과정의 감소(예: 산화된 단백질을 제거하지 못하거나 산화된 DNA를 충분히 빠르게 복구하지 못하는 경우)로 인해 발생할 수도 있으며, 특정 질병에서 두 가지 모두 발생할 수 있습니다.
바이오마커: 신체 또는 그 산물에서 측정할 수 있고 결과 또는 질병의 발생에 영향을 미치거나 예측할 수 있는 모든 물질, 구조 또는 과정으로 정의할 수 있습니다54.
What are ROS, antioxidants and oxidative damage?
One problem that underlies the measurement of ROS and oxidative damage and the use of ‘antioxidants’ is the lack of precision in the use of these terms. ROS is an abbreviation that covers a wide range of chemical species with different properties, reactivities and interactions (Box 1, Table 1). For example, one important reactive species found in biology, the superoxide radical anion (O2•−), is formed by the one-electron reduction of oxygen (O2). In itself, O2•− is not very reactive, except with another radical nitric oxide (•NO) to form peroxynitrite11, or with Fe–S clusters in proteins12. Similarly, hydrogen peroxide (H2O2), formed by various oxidase enzymes1,4 and by the action of superoxide dismutase (SOD), is poorly reactive, which allows its use as an important signalling molecule in vivo2,4. Nevertheless, in the presence of ferrous or cuprous ions, H2O2 forms the extremely reactive hydroxyl radical (•OH) by Fenton chemistry: •OH reacts non-specifically and essentially instantaneously with any nearby biomolecule (Table 1)1,13. The availability of transition metal ions to catalyse Fenton chemistry is carefully controlled in vivo1, but these can be released by tissue injury or when certain proteins with Fe–S clusters encounter O2•− (refs. 1,12,14). Their importance in vivo has recently been underscored by the growing literature on ferroptosis, a form of cell death involving ‘catalytic’ iron ions15. H2O2 is a substrate for haem peroxidases such as myeloperoxidase, generating further reactive species such as HOCl (Table 1). Despite its poor overall reactivity, H2O2 can selectively oxidize some methionine (Met) and cysteine (Cys) residues16,17 in certain proteins.
A (far from complete) list of the physicochemical properties of the most common ROS encountered in biology is given in Table 1, which provides insights into what reactions might be plausible in vivo when these species are generated. What should also be evident is that ‘reactive’ is highly context dependent, because the reactivity of different ROS varies over a wide scale, as do their lifespans, ability to diffuse and potential to generate further downstream reactive species. In short, not all ROS are the same. The generalization ‘ROS’, although widely used (including in this paper!) does not give information about the actual chemical species causing the observed effect. Recommendation 1: wherever possible, the actual chemical species involved in a biological process should be stated and consideration given to whether the observed effect is compatible with its reactivity, lifespan, products generated and fate in vivo. If this is not possible, caveats about use of the term ‘ROS’ should be discussed.
A wide range of antioxidants is present in biology. These include enzymes and small molecules that react with individual ROS to decrease oxidative damage and/or modulate redox signalling1,2. As with ‘ROS’, the use of ‘antioxidant’ as a general term can be imprecise and misleading (Box 1). Often the effect of a putative antioxidant on a biological outcome is used to infer a role for a ROS, as if all antioxidants were equivalent. However, each antioxidant has its own specific chemistry and reactivity with different ROS. Furthermore, the major antioxidants in vivo are enzymatic systems such as SOD for O2•−, peroxidases for H2O2, and metal ion sequestration1,14. Most low-molecular-mass compounds commonly employed as ‘antioxidants’ are stoichiometric scavengers of certain ROS and often have modest (if any) reactivity with O2•− or H2O2. For example, N-acetylcysteine (NAC) is a widely used ‘antioxidant’ but it has other (and sometimes more important18) modes of action. NAC can indeed scavenge some ROS in vitro, but not others, most notably not H2O2 (ref. 18). It can also increase the cellular Cys pool and thereby enhance glutathione (GSH) levels, generate H2S, and directly cleave protein disulphides18. Low-molecular-mass compounds that do act as antioxidants in vivo include vitamin E, which scavenges lipid peroxyl radicals19. Sometimes ‘•OH scavengers’ are used to infer a role for this ROS yet they can rarely, if ever, achieve a sufficiently high concentration to prevent the effectively instantaneous reaction of •OH with biomolecules1,7,13. Consequently, many of the biological effects assigned to ‘antioxidants’, especially NAC, are due to other effects. Other agents often used as ‘antioxidants’, such as TEMPO/TEMPOL, mito-TEMPO and porphyrin-based ‘SOD mimetics’, undergo complex redox reactions in vivo and are better described as ‘redox modulators’ rather than ‘antioxidants’ or ‘O2•− scavengers’1,20,21. Recommendation 2: for an intervention to be attributed to an antioxidant activity, the particular chemical species targeted by the ‘antioxidant’ needs to be made explicit. It should be recognized that low-molecular-mass ‘antioxidants’ are unlikely to act by scavenging H2O2. The specificity, rate constant, location and concentration of the antioxidant within the cell should render an antioxidant effect chemically plausible. Wherever possible the activity of the antioxidant should be confirmed by measuring a decrease in oxidative damage.
A key procedure to attributing oxidative damage, or activation of a redox signalling pathway, to a particular ROS can be by selective generation of the ROS in a biological context. This can be done by using redox cycling compounds such as paraquat (PQ) or quinones to generate O2•−, or MitoPQ to generate O2•− within mitochondria1,22. Of course, increased O2•− generation will also increase H2O2 production by O2•− dismutation. Glucose oxidase can be used to generate H2O2 directly in vitro, while the regulated generation of H2O2 within cells can be achieved using genetically expressed D-amino acid oxidase, an enzyme that generates H2O2 as it oxidizes D-amino acids23. It can be targeted to different sites in the cell, and the flux regulated by varying the added concentration of its substrate, D-alanine23. NADPH oxidase (NOX) enzymes are important sources of O2•−and H2O2 for redox signalling, as well as oxidative damage9,24, and modulation of their activity is an important approach to understanding these processes. A number of fairly specific inhibitors of NOX enzymes have been described24. However, the use of compounds such as apocynin and diphenyleneiodonium as ‘NOX inhibitors’ is still widespread, even though their lack of specificity is well established1,24. Recommendation 3: we recommend the use of PQ, quinones and MitoPQ for selective generation of O2•− and the cellular expression of D-amino acid oxidase for controlled generation of H2O2. Avoid the use of inhibition of a phenomenon by apocynin or diphenyleneiodonium as sole evidence for a role of NOX enzymes, or at least discuss their lack of specificity. Specific inhibitors24 or deletion or knockdown of NOX components should be used to identify their roles.
ROS, 항산화제, 산화적 손상이란 무엇인가요?
ROS와
산화적 손상을 측정하고
'항산화제'를 사용하는 데 있어
한 가지 문제는 이러한 용어의 사용의 정확성이 부족하다는 것입니다.
ROS는
다양한 특성, 반응성 및 상호 작용을 가진 광범위한
https://link.springer.com/article/10.1007/s40828-019-0101-8
예를 들어
생물학에서 발견되는 중요한 반응성 종 중 하나인
슈퍼옥사이드 라디칼 음이온(O2--)은
산소(O2)의 1전자 환원에 의해 형성됩니다.
O2--는
다른 라디칼 산화질소(-NO)와 결합하여
퍼옥시니트라이트11를 형성하거나
단백질의 Fe-S 클러스터12와 결합하는 경우를 제외하고는
그 자체로는 반응성이 크지 않습니다.
마찬가지로
슈퍼옥사이드 디스뮤타제(SOD)의 작용에 의해 형성되는
과산화수소(H2O2)는
반응성이 낮아서 생체 내에서
그럼에도 불구하고
철 이온이나 구리 이온이 존재할 경우
H2O2는 펜톤 화학에 의해
반응성이 매우 높은
하이드 록실 라디칼(-OH)을 형성합니다:
-OH는
주변의 모든 생체 분자와
비특이적이고 본질적으로 즉각적으로 반응합니다(표 1)1,13).
펜톤 화학을 촉매하는
전이 금속 이온의 가용성은
생체 내에서 신중하게 제어되지만1,
조직 손상이나 Fe-S 클러스터를 가진 특정 단백질이
O2--를 만나면 방출될 수 있습니다(참조 1,12,14).
최근 '촉매성' 철 이온과 관련된
세포 사멸의 한 형태인 페로펩토시스에 대한 문헌이 증가하면서
생체 내에서의 중요성이 강조되고 있습니다15.
H2O2는
미엘로퍼옥시다아제와 같은
혈액 과산화효소의 기질로,
HOCl과 같은 반응성 종을 추가로 생성합니다(표 1).
전반적인 반응성은 낮지만,
H2O2는 특정 단백질의 일부
메티오닌(Met) 및 시스테인(Cys) 잔기16,17를
선택적으로 산화시킬 수 있습니다.
생물학에서 가장 흔하게 접하는
ROS의 물리화학적 특성 목록은 표 1에 나와 있으며,
이를 통해 생체 내에서 생성될 때
어떤 반응이 일어날 수 있는지에 대한 통찰력을 얻을 수 있습니다.
또한 분명히 알아야 할 것은
'반응성'은 상황에 따라 크게 달라진다는 점입니다.
다양한 ROS의 반응성은
수명, 확산 능력 및 후속 반응성 종을 생성할 수 있는 잠재력과 마찬가지로
광범위한 범위에서 다양하기 때문입니다.
요컨대,
모든 ROS가 같은 것은 아닙니다.
이 백서를 포함하여 널리 사용되는
'ROS'라는 일반화는
관찰된 효과를 유발하는 실제 화학 종에 대한 정보를 제공하지 않습니다.
권장 사항 1: 가능하면 생물학적 과정에 관여하는 실제 화학 종을 명시하고 관찰된 효과가 반응성, 수명, 생성물 및 생체 내 운명과 양립할 수 있는지 여부를 고려해야 합니다. 이것이 가능하지 않은 경우 'ROS'라는 용어 사용에 대한 주의 사항을 논의해야 합니다.
생물학에는
다양한 항산화제가 존재합니다.
여기에는
개별 ROS와 반응하여
산화적 손상을 줄이거나 산화 환원 신호를 조절하는 효소 및 소분자가 포함됩니다1,2.
'ROS'와 마찬가지로
'항산화제'를 일반적인 용어로 사용하는 것은 부정확하고
오해의 소지가 있을 수 있습니다(박스 1).
종종 추정 항산화제가
생물학적 결과에 미치는 영향을 통해
마치 모든 항산화제가 동일한 역할을 하는 것처럼
ROS의 역할을 유추하는 데 사용됩니다.
그러나
각 항산화제는
고유한 화학적 특성과
다른 ROS와의 반응성을 가지고 있습니다.
또한 생체 내 주요 항산화제는
O2--에 대한 SOD,
H2O2에 대한 과산화효소,
SOD for O2•−,
peroxidases for H2O2, and
metal ion sequestration
일반적으로
'항산화제'로 사용되는 대부분의 저분자량 화합물은
특정 ROS의 화학량론적 제거제이며,
O2-- 또는 H2O2와 반응성이 미미한 경우가 많습니다(있는 경우).
예를 들어,
N-아세틸시스테인(NAC)은
널리 사용되는 '항산화제'이지만
다른 (때로는 더 중요한18) 작용 방식이 있습니다.
NAC는
실제로 시험관 내에서 일부 ROS를 제거할 수 있지만,
다른 것들, 특히 H2O2는 제거하지 못합니다(참고 18).
또한
세포 내 Cys 풀을 증가시켜
글루타치온(GSH) 수치를 높이고,
H2S를 생성하며,
단백질 디설파이드를 직접 분해할 수 있습니다18.
생체 내에서
항산화제로 작용하는 저분자량 화합물로는
지질 과산화 라디칼을 제거하는 비타민 E가 있습니다19.
때때로
'-OH 소거제'가
이 ROS의 역할을 추론하는 데 사용되기도 하지만,
-OH와 생체 분자의 효과적인 순간 반응을 막을 만큼
충분히 높은 농도에 도달하는 경우는 거의 없습니다1,7,13.
따라서
'항산화제', 특
히 NAC에 부여된 많은 생물학적 효과는
다른 효과에 기인합니다.
템포/템폴, 미토 템포, 포르피린 기반 'SOD 모방체'와 같이 '항산화제'로 자주 사용되는 다른 약제는 생체 내에서 복잡한 산화 환원 반응을 거치므로 '항산화제' 또는 '산소 제거제'1,20,21 보다는 '산화 환원 조절제'로 더 잘 설명할 수 있습니다.
권고사항 2: 개입이 항산화 작용에 기인하려면 '항산화제'가 표적으로 삼는 특정 화학 종을 명시해야 합니다.
분자량이 낮은 '항산화제'는
이산화탄소를 제거하여
작용할 가능성이 낮다는 점을 인식해야 합니다.
세포 내 항산화제의 특이성,
속도 상수, 위치 및 농도는
항산화 효과를 화학적으로 그럴듯하게 만들어야 합니다.
가능하면
산화적 손상의 감소를 측정하여
항산화제의 활성을 확인해야 합니다.
산화적 손상 또는 산화 환원 신호 경로의 활성화를
특정 ROS에 기인하는 핵심 절차는
생물학적 맥락에서 ROS를 선택적으로 생성하는 것입니다.
이는
파라콰트(PQ) 또는 퀴논과 같은
산화 환원 순환 화합물을 사용하여
O2--를 생성하거나 미토콘드리아 내에서 MitoPQ를 생성하여1,22 O2--를 생성함으로써
이루어질 수 있습니다.
물론,
O2-- 생성이 증가하면
O2-- 돌연변이에 의한
H2O2 생성도 증가합니다.
포도당 산화효소는
시험관 내에서 직접 H2O2를 생성하는 데 사용할 수 있으며,
세포 내에서 조절된 H2O2 생성은 유전적으로 발현된
D-아미노산을 산화하여 H2O2를 생성하는 효소인
D-아미노산 산화효소23를 사용하여 달성할 수 있습니다.
이 효소는
세포의 여러 부위를 표적으로 삼을 수 있으며,
기질인 D-알라닌의 첨가 농도를 변화시킴으로써
플럭스를 조절할 수 있습니다23.
NADPH 산화효소(NOX) 효소는
산화 환원 신호뿐만 아니라
산화적 손상을 위한 O2 및 H2O2의 중요한 공급원이며9,24,
활성 조절은 이러한 과정을 이해하는 데 중요한 접근 방식입니다.
상당히 구체적인 NOX 효소 억제제가 많이 설명되어 있습니다24.
그러나
아포시닌 및 디페닐렌리오도늄과 같은 화합물을
'NOX 억제제'로 사용하는 것은
그 특이성이 부족하다는 것이 잘 알려져 있음에도 불구하고 여전히 널리 퍼져 있습니다1,24.
권장 사항 3: 선택적 산소 발생을 위해 PQ, 퀴논 및 MitoPQ를 사용하고, H2O2 발생을 제어하기 위해 D-아미노산 산화효소의 세포 발현을 권장합니다. 아포시닌 또는 디페닐렌리오도늄에 의한 현상 억제를 NOX 효소의 역할에 대한 유일한 증거로 사용하지 않거나 적어도 그 특이성 부족에 대해 논의해야 합니다. 특정 억제제24 또는 NOX 성분의 삭제 또는 녹다운을 사용하여 그 역할을 확인해야 합니다.
General principles of measurement of ROS and oxidative damage
When investigating ROS in biological systems, it is important to detect and quantify the ROS of interest. This can be done using electron paramagnetic (spin) resonance (EPR/ESR), various probe molecules or by measuring oxidative modifications (‘oxidative damage’, Box 1) caused by the ROS1. Most ROS probes capture only a small percentage of any ROS formed. Indeed, if the probe reacted with most of the ROS generated this would perturb the system and affect experimental results (for example, inhibition of oxidative damage or interference with redox signalling). However, it is important that the percentage capture remains approximately constant over different rates of production of the ROS in question.
Oxidative damage can take many forms; the chemical processes by which it arises from a particular ROS and how it is assessed and quantified are complex. Furthermore, the final level of any oxidative damage biomarker measured is the difference between its rate of production and its removal by repair, degradation, excretion or diffusion. Recommendation 4: when oxidative damage levels to any biomolecule are presented, the chemical processes by which they arise and the methods used to quantify them should be made explicit. The impact of repair and clearance on the final levels measured should be considered and discussed.
생물학적 시스템에서 ROS를 조사할 때는 관심 있는 ROS를 감지하고 정량화하는 것이 중요합니다. 이는 전자 상자성(스핀) 공명(EPR/ESR), 다양한 프로브 분자를 사용하거나 ROS1로 인한 산화적 변형('산화적 손상', 박스 1)을 측정하여 수행할 수 있습니다. 대부분의 ROS 프로브는 형성된 ROS의 일부만 포착합니다. 실제로 프로브가 생성된 대부분의 ROS와 반응하면 시스템이 교란되어 실험 결과에 영향을 미칠 수 있습니다(예: 산화 손상 억제 또는 산화 환원 신호 간섭). 그러나 포집 비율은 해당 ROS의 다양한 생성 속도에 걸쳐 거의 일정하게 유지되는 것이 중요합니다.
산화 손상은
다양한 형태로 나타날 수 있으며,
특정 ROS에서 발생하는 화학적 과정과 이를 평가하고 정량화하는 방법은 복잡합니다.
또한 측정된 산화적 손상 바이오마커의 최종 수준은
생성 속도와 복구, 분해, 배설 또는 확산에 의한 제거율 간의 차이입니다.
권장 사항 4: 생체 분자에 대한 산화적 손상 수치를 제시할 때는 그 수치가 발생하는 화학적 과정과 이를 정량화하는 데 사용된 방법을 명시해야 합니다. 수리 및 제거가 최종 측정 수준에 미치는 영향을 고려하고 논의해야 합니다.
Guidelines and limitations of the detection and measurement of ROS
Consideration of ROS, antioxidants and oxidative damage as monolithic concepts limits the precision and interpretation of experiments and glosses over the need to establish precise molecular mechanisms. To put these precepts into practice requires measurement of specific ROS and/or oxidative damage products, as well as the effects of antioxidants. This is a major practical challenge, because most ROS are short lived (lifespans of milliseconds or less) and their steady-state levels are low (picomolar to low micromolar) and alter rapidly, because they are affected by continuously varying rates of generation, chemical reaction and diffusion.
In simple in vitro systems it is possible to detect several ROS (Table 2). For example, O2•− production can be monitored by the reduction of cytochrome c, and its selectivity assessed by inhibition by added SOD. However, even such a ‘simple’ system can be surprisingly complex. For example, semiquinones can reduce cytochrome c in a reaction inhibited by SOD25. The bottom line is that all methods used to assess ROS are susceptible to artefact, and appropriate controls are required to be certain of the species and amounts measured. Hence, it is important to corroborate measurements with ‘orthogonal techniques’ that rely on an alternative approach using a different detection method to avoid method-specific artefacts. These complexities are magnified when one attempts to measure ROS in cells. Commonly used cell culture conditions promote oxidative damage due to both limited antioxidants in the medium and high O2 concentrations relative to those in vivo26. Consequently, cultured cells generate more ROS than these cells would in vivo.
ROS 검출 및 측정의 가이드라인과 한계점
ROS, 항산화제 및 산화적 손상을 단일 개념으로 간주하면 실험의 정밀도와 해석이 제한되고 정확한 분자 메커니즘을 확립해야 할 필요성을 간과하게 됩니다. 이러한 교훈을 실천에 옮기려면 항산화제의 효과뿐만 아니라 특정 ROS 및/또는 산화적 손상 생성물의 측정이 필요합니다. 대부분의 ROS는 수명이 짧고(수명이 밀리초 이하) 정상 상태 수준이 낮으며(피코몰~저마이크로몰) 지속적으로 변화하는 생성 속도, 화학 반응 및 확산의 영향을 받기 때문에 빠르게 변화하기 때문에 이는 실용적인 주요 과제입니다.
간단한 체외 시스템에서는 여러 ROS를 감지할 수 있습니다(표 2). 예를 들어, 산소 발생은 사이토크롬 c의 감소로 모니터링할 수 있으며, 선택성은 첨가된 SOD에 의한 억제로 평가할 수 있습니다. 그러나 이러한 '단순한' 시스템도 의외로 복잡할 수 있습니다. 예를 들어, 세미퀴논은 SOD25에 의해 억제된 반응에서 시토크롬 c를 감소시킬 수 있습니다. 결론은 ROS를 평가하는 데 사용되는 모든 방법은 인공물에 영향을 받기 쉬우며, 측정되는 종과 양을 확실히 하기 위해 적절한 제어가 필요하다는 것입니다. 따라서 방법별 인공물을 피하기 위해 다른 검출 방법을 사용하는 대체 접근법에 의존하는 '직교 기법'으로 측정값을 확증하는 것이 중요합니다. 이러한 복잡성은 세포에서 ROS를 측정하려고 할 때 더욱 커집니다. 일반적으로 사용되는 세포 배양 조건은 배지 내 항산화제가 제한적이고 생체에 비해 높은 산소 농도로 인해 산화적 손상을 촉진합니다26. 결과적으로 배양된 세포는 생체 내 세포보다 더 많은 ROS를 생성합니다.
Table 2 Some recommended approaches for detection and quantification of ROS in different biological contexts
Recommendation 5: use commercial kits only if the actual species being measured and the method of detection are explained in the kit materials, are chemically plausible and the limitations are understood. The use of commercial kits without such information is strongly discouraged. To avoid method-specific artefacts, confirm results using techniques that rely on different principles of detection.
권장 사항 5: 키트 자료에 실제 측정 대상 종과 검출 방법이 설명되어 있고 화학적으로 타당하며 제한 사항을 이해한 경우에만 상업용 키트를 사용하세요. 이러한 정보가 없는 상용 키트의 사용은 강력히 권장하지 않습니다. 방법별 인공물을 피하려면 다른 검출 원리에 의존하는 기술을 사용하여 결과를 확인하세요.
Small-molecule fluorescent probes are frequently used to assess ROS within cells. In some cases, often involving kits, a lack of description of the chemical reactivity or structures of these probes makes it difficult to interpret results and therefore such probes should be avoided. Even for probes of known structure there can be concerns. Consider the widely used fluorescent probe 2',7'-dichlorodihydrofluorescein (DCFH), usually administered in its diacetate (DCFH-DA) form, which enters cells readily. DCFH is oxidized to the fluorescent product 2',7'-dichlorofluorescein (DCF) by several ROS, and so it is not specific for any particular ROS6,7. DCFH is not oxidized directly by H2O2 (which it is often claimed to detect), but only after H2O2 is converted to more reactive species by redox-active metals or by haem proteins such as cytochrome c or peroxidases. Furthermore, the oxidation of DCFH and fluorescence of DCF are sensitive to local O2 levels and pH, and fluorescence yield may not be linear with increased ROS levels27,28,29. This is not to say that DCFH, and other non-specific fluorescent probes such as dihydrorhodamine, should never be used, but their limitations (selectivity, problems of quantification, linearity of response and susceptibility to artefact) should be understood and results interpreted cautiously28. In particular, their response should not be attributed to a specific ROS without detailed controls to validate this, and their use should be restricted to an initial assessment of a change in cellular redox state, to be followed up by a more detailed investigation into mechanism. While many small-molecule and protein fluorescent probes are more selective than DCF, it is always important to validate data by a number of simple controls: does the response change over time and with the amount of biological sample in a plausible manner? Can the effect be replicated by generating the ROS of interest (for example, using PQ for O2•− or D-amino acid oxidase for H2O2)? Do negative controls that should abolish the ROS-generating process (for example, gene knockouts, knockdowns, inhibitors, radical scavengers) respond as expected? Recommendation 6: when using fluorescent ROS probes (especially DCFH-DA), the chemistry involved, the selectivity for particular chemical species and potential artefacts should be made clear and discussed. Wherever possible, controls to show that the response is due to the proposed species should be carried out and orthogonal techniques used to corroborate the conclusion.
Extending measurements of ROS from cells in culture to tissues in vivo or ex vivo is vital. However, in some cases this gap has been addressed by the addition of ‘ROS probes’ to fresh or previously frozen tissue slices or homogenates ex vivo. These measurements may be meaningless, because the very short lifespan of ROS means that any present in vivo will have long disappeared by the time the material is assayed. Furthermore, freezing or homogenization disrupts membranes and alters substrate and ion concentrations (for example, raising levels of Ca2+ or ‘catalytic’ Fe2+)1, such that any ROS production in the tissue slice or homogenate bears no relation to the levels that would have been generated in vivo. There are valid methods available to assess ROS in vivo or in perfused organs, but in these situations the process is either monitored in vivo (for example, see Table 2 for the use of catalase compound I to measure H2O2) or the system is quenched to stabilize the probe for analysis ex vivo. Recommendation 7: measurements of ROS should be carried out in cells, tissues or organs under physiologically relevant conditions in vivo or ex vivo. ROS should not be ‘measured’ in tissue homogenates or cryosections, unless the probe or sensor employed is able to irreversibly capture the reactive species when the cells/tissues/organs are under biologically relevant conditions.
저분자 형광 프로브는 세포 내 ROS를 평가하는 데 자주 사용됩니다. 키트와 관련된 일부 경우, 이러한 프로브의 화학 반응성이나 구조에 대한 설명이 부족하면 결과를 해석하기 어렵기 때문에 이러한 프로브는 피해야 합니다. 구조가 알려진 프로브의 경우에도 우려가 있을 수 있습니다. 널리 사용되는 형광 프로브인 2',7'-디클로로디하이드로플루오레세인(DCFH)은 일반적으로 세포에 쉽게 들어가는 디아세테이트(DCFH-DA) 형태로 투여됩니다. DCFH는 여러 ROS에 의해 형광 생성물인 2',7'-디클로로플루오레세인(DCF)으로 산화되므로 특정 ROS6,7에 특이적이지 않습니다. DCFH는 H2O2에 의해 직접 산화되는 것이 아니라(종종 검출한다고 주장됨) 산화 환원 활성 금속이나 시토크롬 C 또는 과산화효소와 같은 혈액 단백질에 의해 H2O2가 더 반응성이 높은 종으로 전환된 후에만 산화됩니다. 또한, DCFH의 산화와 DCF의 형광은 국소 O2 수준과 pH에 민감하며 형광 수율은 ROS 수준이 증가함에 따라 선형적이지 않을 수 있습니다27,28,29. 그렇다고 DCFH 및 디하이드로호다민과 같은 기타 비특이적 형광 프로브를 절대 사용해서는 안 된다는 것은 아니지만, 그 한계(선택성, 정량화 문제, 반응의 선형성 및 인공물에 대한 민감성)를 이해하고 결과를 신중하게 해석해야 합니다28. 특히, 이를 검증하기 위한 세부적인 대조군 없이 특정 ROS에 의한 반응으로 간주해서는 안 되며, 세포 산화 환원 상태의 변화에 대한 초기 평가로 제한하여 사용하고 이후 메커니즘에 대한 보다 자세한 조사를 수행해야 합니다. 많은 저분자 및 단백질 형광 프로브가 DCF보다 더 선택적이지만, 항상 여러 가지 간단한 제어를 통해 데이터를 검증하는 것이 중요합니다. 시간이 지남에 따라 그리고 생물학적 샘플의 양에 따라 반응이 그럴듯한 방식으로 변화하는가? 관심 있는 ROS를 생성하여 효과를 재현할 수 있는가(예: O2에 PQ 또는 H2O2에 D-아미노산 산화효소 사용)? ROS 생성 과정을 폐지해야 하는 음성 대조군(예: 유전자 녹아웃, 녹다운, 억제제, 라디칼 제거제)이 예상대로 반응하는가?
권장 사항 6: 형광 ROS 프로브(특히 DCFH-DA)를 사용할 때는 관련된 화학 물질, 특정 화학 종에 대한 선택성 및 잠재적 인공물을 명확히 밝히고 논의해야 합니다. 가능한 경우, 반응이 제안된 종에 의한 것임을 보여주기 위한 제어를 수행하고 결론을 확증하기 위해 직교 기법을 사용해야 합니다.
배양 중인 세포에서 생체 내 또는 생체 외 조직으로 ROS 측정을 확장하는 것이 중요합니다. 그러나 경우에 따라 신선하거나 이전에 냉동한 조직 슬라이스 또는 생체 외 균질물에 'ROS 프로브'를 추가하여 이 격차를 해결하기도 합니다. ROS의 수명이 매우 짧기 때문에 생체 내에 존재하는 물질은 분석할 때쯤이면 이미 사라졌을 것이므로 이러한 측정은 의미가 없을 수 있습니다. 또한 동결 또는 균질화는 막을 파괴하고 기질 및 이온 농도(예: Ca2+ 또는 '촉매' Fe2+ 수준 상승)1를 변화시켜 조직 슬라이스 또는 균질화에서 생성된 ROS는 생체 내에서 생성된 수준과 관련이 없습니다. 생체 내 또는 관류된 장기에서 ROS를 평가할 수 있는 유효한 방법이 있지만 이러한 상황에서는 생체 내에서 프로세스를 모니터링하거나(예: 카탈라아제 화합물 I을 사용하여 H2O2를 측정하는 경우 표 2 참조) 생체 외 분석을 위해 프로브를 안정화하기 위해 시스템을 냉각합니다.
권장 사항 7: ROS 측정은 생체 내 또는 생체 외의 생리학적 관련 조건에서 세포, 조직 또는 기관에서 수행해야 합니다. 세포/조직/기관이 생물학적으로 관련된 조건에 있을 때 사용된 프로브 또는 센서가 반응성 종을 비가역적으로 포착할 수 있는 경우가 아니라면 조직 균질물 또는 동결 절편에서 ROS를 '측정'해서는 안 됩니다.
Direct measurement of ROS
Here we outline what we consider to be, currently, the best approaches to the measurement of commonly encountered ROS.
Superoxide
In simple systems O2•− can be measured in a number of ways, such as by the SOD-inhibitable reduction of cytochrome c (ref. 25). The generation of O2•− can also be assessed by spin trapping followed by EPR, which has the benefit of direct detection of the radical1. The Fe–S cluster in aconitase is inactivated by O2•−, and by other ROS, but its interaction with O2•− is fast, reasonably specific and reversible, making it a good indicator for O2•− in mitochondria30. The chemiluminescent ‘superoxide probes’ luminol and lucigenin are widely used to ‘detect O2•−’, but interpretation of such data is difficult because these probes generate radicals that produce O2•− themselves; they do not react with O2•− directly31,32.
Recommendation 8: the use of luminol and lucigenin to ‘detect O2•−’ should be discouraged, but they can be used as general indicators of increased ROS production. SOD-sensitive reduction of cytochrome c in vitro and aconitase inactivation within mitochondria are better strategies.
In cells, O2•− is often detected by measuring the fluorescence arising from oxidation of dihydroethidium (sometimes called hydroethidine (HE)), or mitochondria-targeted HE (MitoSOX). Unfortunately, detection by fluorescence is misleading because these probes form both ethidium (E+), a non-specific oxidation product, and the O2•−-specific product 2-hydroxyethidium. Because these two products have overlapping fluorescence spectra, it is hard to differentiate the contribution of non-specific oxidation and O2•−-dependent oxidation (if any) to the overall fluorescence33. Accurate quantification of the 2-hydroxyethidium product can be achieved using liquid chromatography–mass spectrometry (LC–MS)33. Another factor that should be considered is the extent of cellular uptake of HE/MitoSOX and the intracellular concentrations of these and their multiple products. Furthermore, HE oxidation products intercalate into DNA, greatly enhancing their fluorescence and creating another artefact. NeoD and MitoNeoD contain a modified HE that does not intercalate into DNA34.
Mitochondrially accumulated O2•−probes, such as MitoSOX, are often used to ‘detect O2•−’ within mitochondria. When using these probes, and others that have positive charges or generate positively charged species (including 2-hydroxyethidium and ethidium), it is important to remember that probe accumulation is dependent on plasma and mitochondrial membrane potentials and mitochondrial size, shape and mass35. Furthermore, fluorescence can be quenched when these are present at high concentrations in mitochondria36.
ROS의 직접 측정
여기에서는 현재 일반적으로 발생하는 ROS를 측정하는 가장 좋은 방법이라고 생각되는 방법을 간략하게 설명합니다.
슈퍼옥사이드
간단한 시스템에서 활성산소(O2--)는 SOD에 의해 억제되는 사이토크롬 c의 감소와 같은 여러 가지 방법으로 측정할 수 있습니다(참조 25). 또한 스핀 트래핑에 이어 라디칼을 직접 검출할 수 있는 이점이 있는 EPR을 통해 O2--의 생성을 평가할 수도 있습니다1. 아코니타제의 Fe-S 클러스터는 O2-- 및 다른 ROS에 의해 비활성화되지만, O2--와의 상호작용은 빠르고 상당히 특이적이며 가역적이어서 미토콘드리아에서 O2--에 대한 좋은 지표가 됩니다30. 화학 발광 '슈퍼옥사이드 프로브' 루미놀과 루시게닌은 'O2-- 검출'에 널리 사용되지만, 이러한 프로브는 자체적으로 O2--를 생성하는 라디칼을 생성하고 O2--와 직접 반응하지 않기 때문에 이러한 데이터의 해석이 어렵습니다31,32.
권장 사항 8: 루미놀과 루시게닌을 'O2-- 검출'에 사용하는 것은권장하지 않지만, ROS 생성 증가의 일반적인 지표로 사용할 수 있습니다. 시험관 내에서 SOD에 민감한 사이토크롬 c의 감소와 미토콘드리아 내의 아코니타제 비활성화가 더 나은 전략입니다.
세포에서 O2--는 디하이드로에티듐(하이드로에티딘(HE)이라고도 함)의 산화로 인해 발생하는 형광 또는 미토콘드리아 표적 HE(MitoSOX)를 측정하여 검출하는 경우가 많습니다. 안타깝게도 이러한 프로브는 비특이적 산화 생성물인 에티듐(E+)과 산소 특이적 생성물인 2-하이드록시에티듐을 모두 형성하기 때문에 형광을 통한 검출은 오해의 소지가 있습니다. 이 두 생성물은 형광 스펙트럼이 겹치기 때문에 전체 형광에 대한 비특이적 산화와 O2-- 의존적 산화(있는 경우)의 기여도를 구분하기 어렵습니다33. 액체 크로마토그래피-질량 분석법(LC-MS)33을 사용하여 2-하이드록시 에티듐 생성물을 정확하게 정량화할 수 있습니다. 고려해야 할 또 다른 요소는 HE/MitoSOX의 세포 내 흡수 정도와 이들 및 여러 생성물의 세포 내 농도입니다. 또한 HE 산화 생성물은 DNA에 인터칼레이팅되어 형광을 크게 향상시키고 또 다른 인공물을 생성합니다. NeoD와 MitoNeoD에는 DNA로 인터칼레이팅되지 않는 변형된 HE가 포함되어 있습니다34.
미토콘드리아에 축적된 O2-- 프로브는 종종 미토콘드리아 내에서 'O2--를 검출'하는 데 사용됩니다(예: MitoSOX). 이러한 프로브와 양전하를 띠거나 양전하를 띠는 종(2-하이드록시 에티듐 및 에티듐 포함)을 생성하는 다른 프로브를 사용할 때는 프로브 축적은 혈장 및 미토콘드리아 막 전위와 미토콘드리아 크기, 모양 및 질량에 따라 달라진다는 점을 기억하는 것이 중요합니다35. 또한 형광은 미토콘드리아에 고농도로 존재할 때 소멸될 수 있습니다36.
Recommendation 9: use only HE or MitoSOX probes to detect O2•−by simple fluorescence measurements when the product has been independently validated as 2-hydroxyethidium. Fluorescence measurements with probes such as dihydroethidium and MitoSOX33 should be conducted using the lowest probe concentration possible, and must include controls for changes in plasma and mitochondrial membrane potentials and mitochondrial mass and morphology, such as normalization to a similar membrane-potential-responsive, but redox-insensitive, probe. LC–MS methods, which measure all modified species33, should be performed when possible.
권장 사항 9: 제품이 2-하이드록시에티듐으로 독립적으로 검증된 경우 간단한 형광 측정을 통해 O2를 검출하는 데 HE 또는 MitoSOX 프로브만 사용하세요. 디하이드록시에티듐 및 MitoSOX33과 같은 프로브를 사용한 형광 측정은 가능한 가장 낮은 프로브 농도를 사용하여 수행해야 하며, 혈장 및 미토콘드리아 막 전위와 미토콘드리아 질량 및 형태 변화에 대한 제어(예: 유사한 막 전위 반응성이 있지만 산화 환원에 민감하지 않은 프로브로의 정상화)가 포함되어야 합니다. 가능한 경우 모든 변형된 종33을 측정하는 LC-MS 방법을 수행해야 합니다.
Hydrogen peroxide
In simple systems, H2O2 can be measured by horseradish peroxidase (HRP)-oxidizing substrates, one frequently used being Amplex Red. These methods can be interfered with by other HRP substrates (for example, ascorbate and NAC)1 and by O2•− (which can inactivate HRP), the latter preventable by the addition of SOD37. Since H2O2 can cross membranes directly or via aquaporins, this system can also be used to measure H2O2 release from cells. However, please be aware that this release reflects the balance between H2O2 production, removal by intracellular enzymes and the rate of diffusion out of the cell.
Within cells, H2O2 detection by phenylboronate-based probes is more reliable38 although these may lack sufficient sensitivity because they react only slowly with H2O2, which can make it difficult to detect small or localized changes in H2O2 levels39. However, recent studies suggest that borinic acids, which react more rapidly with H2O2, may be more sensitive detectors40. The mechanism of oxidation of phenylboronates to phenols requires a two-electron oxidant, such as H2O2. Because H2O2 is typically generated at higher concentrations than other ROS, boronate probes can be selective for H2O2 detection subject to proper controls39,40. However, boronate probes react with ONOO−/ONOOH or HOCl much more rapidly than they do with H2O2 which can sometimes complicate measurements, and orthogonal approaches or the use of inhibitors can aid validation41. For example, H2O2- and peroxynitrite-dependent signals can be distinguished using nitric oxide synthase (NOS) inhibitors and catalase38,39,41,42.
Genetically encoded fluorescent protein sensors have provided major advances in cellular H2O2 detection43,44,45,46. These probes contain a dithiol switch that changes the overall fluorescence of the probe depending on its oxidation status. High sensitivity and specificity for H2O2 have been achieved by coupling a redox-sensitive green fluorescent protein (GFP) mutant to a H2O2-sensitive thiol protein, such as oxyR (HyPer series), or to a peroxidase such as Orp1 or TSA2 (roGFP2-based probes). HyPer7 and roGFP2 coupled to a peroxiredoxin provide the highest sensitivity44,45. While HyPer7- and roGFP2-based probes are pH stable, earlier versions of HyPer are not and require expression of a control probe (SypHer) to control for signal changes due to variation in pH43. Imaging analysis by fluorescence microscopy is normally employed, but fluorescence plate readers can also be used. The redox status measured represents a balance between the rate of oxidation and re-reduction of the probes by cellular reductants, including glutaredoxin/GSH and thioredoxin, permitting real-time, live-cell assessments of redox state. Because excitation wavelengths of both reduced and oxidized probes are used, the probes are ratiometric and the output is not dependent on the level of protein probe expression. By incorporation of appropriate targeting gene sequences, these probes can be directed to different cell compartments, including mitochondria, microtubules, endoplasmic reticulum, nucleus and cytoplasm43,44,45,46. Hence, subcellular regions of interest can be studied and the probe then calibrated at the end by full reduction (2 mM dithiothreitol), washout and full oxidation (2 mM t-butylhydroperoxide)44,45. This calibration yields a measure of oxidation percentage, permitting comparisons across experiments and among subcellular compartments44,45. These probes have been expressed in transgenic animals to provide useful assessments of in vivo H2O2 generations46,47. Plasmid transfection of viral vectors can be used with cultured cells, and targeted roGFP2 probes are available commercially (www.addgene.com).
In most experiments the H2O2 probes are expressed as free proteins that distribute within the cell. Nevertheless, given uncertainties about intracellular H2O2 diffusion distances, it is still unclear what resolution is needed to understand subcellular H2O2 distribution. Therefore, tethering H2O2 probes to sub-compartmental locations such as protein complexes or organelle contact sites is an important approach.
과산화수소
간단한 시스템에서 H2O2는 양 고추냉이 과산화효소(HRP)를 산화시키는 기질로 측정할 수 있으며, 자주 사용되는 기질 중 하나는 Amplex Red입니다. 이러한 방법은 다른 HRP 기질(예: 아스코르브산염 및 NAC)1과 O2--(HRP를 비활성화할 수 있음)에 의해 방해를 받을 수 있으며, 후자는 SOD37을 추가하여 방지할 수 있습니다. H2O2는 직접 또는 아쿠아포린을 통해 세포막을 통과할 수 있으므로 이 시스템을 사용하여 세포에서 H2O2 방출을 측정할 수도 있습니다. 그러나 이 방출은 H2O2 생성, 세포 내 효소에 의한 제거 및 세포 밖으로의 확산 속도 사이의 균형을 반영한다는 점에 유의하세요.
세포 내에서 페닐보로네이트 기반 프로브에 의한 H2O2 검출이 더 신뢰할 수 있지만38, 이는 H2O2와 천천히 반응하기 때문에 충분한 감도가 부족하여 H2O2 수준의 작거나 국소적인 변화를 감지하기 어려울 수 있습니다39. 그러나 최근 연구에 따르면 H2O2와 더 빠르게 반응하는 붕산이 더 민감한 검출기가 될 수 있다고 합니다40. 페닐보로네이트가 페놀로 산화되는 메커니즘에는 H2O2와 같은 2전자 산화제가 필요합니다. H2O2는 일반적으로 다른 ROS보다 높은 농도에서 생성되기 때문에 붕산염 프로브는 적절한 제어에 따라 H2O2 검출에 선택적으로 사용될 수 있습니다39,40. 그러나 붕산염 프로브는 H2O2보다 ONOO-/ONOOH 또는 HOCl과 훨씬 더 빠르게 반응하여 측정을 복잡하게 만들 수 있으며, 직교 접근법이나 억제제를 사용하면 검증을 도울 수 있습니다41. 예를 들어, 산화질소 합성효소(NOS) 억제제와 카탈라아제38,39,41,42를 사용하여 H2O2 및 과산화아질산염 의존 신호를 구분할 수 있습니다.
유전자 암호화 형광 단백질 센서는 세포 내 H2O2 검출에 큰 발전을 가져왔습니다43,44,45,46. 이러한 프로브에는 산화 상태에 따라 프로브의 전반적인 형광을 변화시키는 디티올 스위치가 포함되어 있습니다. 산화 환원에 민감한 녹색 형광 단백질(GFP) 돌연변이를 oxyR(HyPer 시리즈)과 같은 H2O2 민감 티올 단백질 또는 Orp1 또는 TSA2(roGFP2 기반 프로브)와 같은 퍼옥시다제에 결합함으로써 H2O2에 대한 높은 감도와 특이성을 달성할 수 있습니다. HyPer7 및 roGFP2를 퍼옥시레독신에 결합하면 가장 높은 감도를 제공합니다44,45. HyPer7 및 roGFP2 기반 프로브는 pH 안정적이지만, 이전 버전의 HyPer는 그렇지 않기 때문에 pH 변화로 인한 신호 변화를 제어하기 위해 제어 프로브(SypHer)의 발현이 필요합니다43. 형광 현미경을 통한 영상 분석이 일반적으로 사용되지만 형광 플레이트 판독기를 사용할 수도 있습니다. 측정된 산화 환원 상태는 글루타레독신/GSH 및 티오레독신을 포함한 세포 환원제에 의한 프로브의 산화 및 재환원 속도 사이의 균형을 나타내므로 실시간으로 살아있는 세포의 산화 환원 상태를 평가할 수 있습니다. 환원 및 산화 프로브의 여기 파장을 모두 사용하기 때문에 프로브는 비율 측정이 가능하며 단백질 프로브 발현 수준에 따라 출력이 달라지지 않습니다. 적절한 표적 유전자 서열을 통합함으로써 이러한 프로브는 미토콘드리아, 미세소관, 소포체, 핵 및 세포질43,44,45,46을 포함한 다양한 세포 구획으로 향하게 할 수 있습니다. 따라서 관심 있는 하위 세포 영역을 연구한 다음 프로브를 완전 환원(2mM 디티오트레이톨), 세척 및 완전 산화(2mM t-부틸하이드로퍼옥사이드)44,45로 마지막에 보정할 수 있습니다. 이 보정을 통해 산화 백분율을 측정하여 실험 전반과 세포 내 구획 간 비교가 가능합니다44,45. 이러한 프로브는 형질 전환 동물에서 발현되어 생체 내 H2O2 생성에 대한 유용한 평가를 제공합니다46,47. 바이러스 벡터의 플라스미드 감염은 배양된 세포와 함께 사용할 수 있으며, 표적화된 roGFP2 프로브는 상업적으로 이용 가능합니다(www.addgene.com).
대부분의 실험에서 H2O2 프로브는 세포 내에 분포하는 유리 단백질로 발현됩니다. 그럼에도 불구하고 세포 내 H2O2 확산 거리에 대한 불확실성을 고려할 때 세포 하부 H2O2 분포를 이해하는 데 필요한 해상도는 여전히 불분명합니다. 따라서 단백질 복합체 또는 세포 소기관 접촉 부위와 같은 하위 구획 위치에 H2O2 프로브를 테더링하는 것이 중요한 접근 방식입니다.
Recommendation 10: genetically encoded fluorescent probes (some of which are commercially available) are currently the most sensitive detectors of H2O2 and we recommend their use in cells and animals if expression is possible. Boronate probes (some of which are also commercially available) are the preferred small-molecule probes, but controls to determine specificity for H2O2 are required and sensitivity is limited for physiological H2O2 levels. Amplex Red with HRP can measure H2O2 release from cells if other reducing agents or peroxidase substrates are absent.
권장 사항 10: 유전자 암호화 형광 프로브(일부는 시판 중)는 현재 가장 민감한 H2O2 검출기이며, 발현이 가능한 경우 세포와 동물에서 사용할 것을 권장합니다. 붕산염 프로브(일부는 시판 중)는 선호되는 저분자 프로브이지만 H2O2에 대한 특이성을 확인하기 위한 제어가 필요하고 생리적 H2O2 수준에 대한 감도가 제한적입니다. 다른 환원제나 퍼옥시다아제 기질이 없는 경우 Amplex Red with HRP는 세포에서 H2O2 방출을 측정할 수 있습니다.
Peroxynitrite
Peroxynitrite (ONOO−) exhibits complex chemistry42,48,49 and itself can oxidize certain biomolecules. A major physiological reaction is with CO2 (Table 1), and hence the CO2/ HCO3− content of biological systems plays a role in determination of the biological impact50 of ONOO−. Products of this reaction include reactive species such as the carbonate radical anion (CO3•−) and the nitrating agent nitrogen dioxide (NO2•) (Table 1), both of which react with many of the general ‘ROS probes’. Peroxynitrite oxidizes boronate-based probes nearly a million times more rapidly than H2O2 and, under the right conditions, these probes can be used to assess ONOO−/ONOOH production42,49. Peroxynitrite has been measured in tissues ex vivo using boronate probes51.
퍼옥시니트라이트
퍼옥시니트라이트(ONOO-)는 복잡한 화학42,48,49을 나타내며 그 자체로 특정 생체 분자를 산화시킬 수 있습니다. 주요 생리적 반응은 CO2와의 반응이며(표 1), 따라서 생물학적 시스템의 CO2/HCO3- 함량은 ONOO-의 생물학적 영향50을 결정하는 데 중요한 역할을 합니다. 이 반응의 생성물에는 탄산염 라디칼 음이온(CO3--)과 질산화제 이산화질소(NO2-)와 같은 반응성 종(표 1)이 포함되며, 이 둘은 일반적인 'ROS 프로브'와 반응하는 경우가 많습니다. 과산화아질산염은 붕산염 기반 프로브를 H2O2보다 거의 백만 배 더 빠르게 산화시키며, 적절한 조건에서 이러한 프로브를 사용하여 ONOO-/ONOOH 생성을 평가할 수 있습니다42,49. 붕산염 프로브를 사용하여 생체 외 조직에서 퍼옥시니트라이트가 측정되었습니다51.
Hypochlorous acid and other reactive halogen species
HOCl, hypobromous acid (HOBr) and some of the chloramines and bromamines derived from them (Table 1) react with most of the general probes used to detect ROS, including DCFH and luminol. However, many of these probes are also substrates of the peroxidases that generate HOCl or HOBr, confounding their use. More specific fluorescent probes for reactive halogen species have been reported and some are commercially available52. A genetically encoded probe for reactive halogen species has been developed, enabling dynamic monitoring of these species both in cell culture and in vivo53.
차아 염소산 및 기타 반응성 할로겐 종
HOCl, 차아 염소산(HOBr) 및 이들에서 파생된 일부 클로라민 및 브로마민(표 1)은 DCFH 및 루미놀을 포함하여 ROS 검출에 사용되는 대부분의 일반 프로브와 반응합니다. 그러나 이러한 프로브 중 다수는 HOCl 또는 HOBr을 생성하는 퍼옥시다아제의 기질이기도 하므로 사용에 혼란을 줄 수 있습니다. 반응성 할로겐 종에 대한 보다 구체적인 형광 프로브가 보고되었으며 일부는 상업적으로 이용 가능합니다52. 반응성 할로겐 종에 대한 유전자 암호화 프로브가 개발되어 세포 배양과 생체 내에서 이러한 종을 동적으로 모니터링할 수 있게 되었습니다53.
Measurement of oxidative damage
The presence of ROS can be inferred by their effects on protein, carbohydrates, nucleic acids and lipids to generate specific compounds which, so long as they cannot be formed by other mechanisms, can be used as ‘biomarkers’ of oxidative damage (Box 1)1,54,55. However, do note that the measured levels of biomarkers represent a balance between the generation and removal of the biomarker (for example, by degradation, diffusion or excretion), plus any artefactual increased levels caused by oxidative damage during isolation or analysis.
산화적 손상 측정
ROS의 존재는 단백질, 탄수화물, 핵산 및 지질에 미치는 영향을 통해 추론할 수 있으며, 다른 메커니즘에 의해 형성될 수 없는 한 특정 화합물을 생성하여 산화 손상의 '바이오마커'로 사용할 수 있습니다(박스 1)1,54,55). 그러나 측정된 바이오마커 수준은 바이오마커의 생성 및 제거(예: 분해, 확산 또는 배설)와 분리 또는 분석 중 산화적 손상으로 인한 인위적인 수준 증가 사이의 균형을 나타낸다는 점에 유의하세요.
Lipid peroxidation
Polyunsaturated fatty acids (PUFAs), are readily oxidized, and hence lipid peroxidation products are widely used to characterize oxidative damage56,57,58. Lipid peroxidation can be initiated by certain ROS and proceed as a random, non-enzymatic (often chain) radical process. However, there are also enzymatic mechanisms (for example, lipoxygenases) available for peroxidation of free PUFAs or PUFA-phospholipids that produce specific signalling products with biological roles. Thus, when measuring lipid peroxidation the focus might be placed on either (1) establishment of increased lipid peroxidation as an example of oxidative damage or (2) identification of individual oxidatively modified lipid molecules acting as signals by selective interaction with certain cellular targets.
In PUFAs, the presence of a double bond adjacent to a methylene group makes the methylene C–H bond weaker and therefore the bis-allylic hydrogen is more susceptible to H• abstraction56,57,58. The carbon-centred radical (L) generated by H• abstraction is stabilized by delocalization over the double bonds. Subsequent reaction with O2 gives a peroxyl radical (LOO) with the formation of a conjugated diene system and a range of peroxides (LOOH). LOO• can react further to yield highly oxidized secondary products, including epoxy-, oxo- or cyclic peroxides56,57,58. Hence, there are multiple end products of lipid peroxidation that show vast chemical heterogeneity and variable stability and polarity, and thus measurement of only a single oxidation product in no way represents the whole process of lipid peroxidation.
Several methods are available to assess ‘general’ lipid peroxidation. In simple model systems (for example, isolated lipoproteins), diene conjugation can be measured by ultraviolet (UV) absorbance but this method is not suitable for use in cells or body fluids because of the presence of interfering UV-absorbing molecules that do not result from lipid peroxidation1. In cells, ‘lipid peroxidation’ can be assessed by changes in the fluorescence of BODIPY conjugated to a peroxidation-sensitive undecanoic acid moiety59. This assay is technically simple but should be interpreted cautiously because BODIPY’s rate of reaction with peroxyl radicals is slower than that of radical-scavenging antioxidants, and hence suppression of BODIPY fluorescence by antioxidants need not always reflect their ability to suppress lipid peroxidation59. Another fluorometric assay for lipid peroxidation employs cis-parinaric acid (PnA), a fatty acid with four conjugated double bonds. Oxidation of PnA disrupts its conjugated system and hence fluorescence. Because PnA may be incorporated into different classes of phospholipid, high-performance liquid chromatography (HPLC) separation provides information on the oxidation of different phospholipids60. However, extrapolation of PnA-based results to endogenous phospholipid oxidation is difficult due to the higher oxidation rate of PnA, its vulnerability to photobleaching and variable metabolic incorporation of PnA into different phospholipids60.
Lipid peroxidation is frequently assessed by the measurement of end products such as α,β-unsaturated hydroxyalkenals61, ideally by MS-based techniques. In particular, 4-hydroxynonenal (HNE) formation has been widely used. Antibodies against the protein adducts formed by HNE are widely available and frequently used in immunostaining of tissues, but it should be realized that different antibodies can detect different epitopes and so give different answers, depending on what amino acid residues the HNE binds to in proteins61,62,63,64.
One minor end product of lipid peroxidation is malondialdehyde (MDA)61, which can also be a useful biomarker if measured by MS techniques. However, the widely used ‘MDA assays’ utilizing thiobarbituric acid-reactive substances (TBARS) are unspecific since TBA generates chromogens from many biomolecules other than MDA1,65. Use of HPLC to separate the ‘real’ TBA–MDA adduct from false chromogens increases specificity but does not eliminate all problems1.
Recommendation 11: application of the simple TBA test (TBARS), or kits based on its use, to cells, tissues or body fluids is not recommended as the only test used for evaluation of oxidative lipid damage because of the low specificity that can result in false-positive results. HPLC-based TBA tests are less prone to artefacts.
The detection of lipid oxidation products has been revolutionized by the development of LC–MS for detailed analysis of oxidized lipid mixtures66. Collection and storage of samples to avoid artefactual peroxidation is key to any lipid peroxidation study, and samples for later analysis should be immediately frozen in liquid nitrogen. Biofluids may require the addition of chemicals (for example, butylated hydroxytoluene) to prevent auto-oxidation during storage1,67. The internal standards used for quantification should be added to samples before solvent extraction. Such LC–MS-based methods have the advantages of high sensitivity, small sample volume requirements and the ability to detect multiple end products of lipid peroxidation. This makes LC–MS protocols the methods of choice for assessment of general lipid peroxidation and identification of individual products, including those with specific signalling functions. However, limitations of available standards may sometimes preclude quantitative analysis of certain products. Scrupulous attention to methodology is required in such studies68.
Prominent among lipid oxidation products that have been quantified by MS-based approaches are the F2-isoprostanes (F2-IsoPs)69. Sixty-four F2-IsoP stereoisomers can be generated from the free-radical-induced, non-enzymatic oxidation of arachidonic acid and can be separated from those that arise from the enzymatic oxidation of arachidonic acid by cyclo-oxygenase enzymes (COX-1/-2). F3- and F4-isoprostanes arise from eicosapentaenoic acid (EPA) and docosahexaenoic acid (DHA), respectively, but have been less well characterized than the F2-isoprostanes. ELISA methods have been developed to quantify one F2-IsoP isomer, 8-iso-PGF2α (also referred to as 15-F2t-IsoP or iPF2α-III), and compared with gas chromatography–MS and LC–tandem MS (LC–MS/MS) methods69,70,71,72,73. In all these studies there was poor agreement between commercially available ELISA kits and MS methods; 8-iso-PGF2α is one of 64 different F2-IsoP isomers generated during arachidonic acid peroxidation, and antibody cross-reactivity between 8-iso-PGF2α and related isomers is challenging. Pre-analysis sample clean-up may allow for more precise measurement of 8-iso-PGF2α by ELISA72,73, but by far the most accurate method for quantification of F2-IsoPs is by LC–MS/MS and is very strongly recommended.
Recommendation 12: F2-IsoPs are a generally accepted biomarker of lipid peroxidation, but it should be realized that they are one of many end products and that the levels of various types can be affected by experimental conditions69. Quantification using ELISA is susceptible to artefact, but sample clean-up may allow measurement of 8-iso-PGF2α by ELISA73. LC–MS/MS with appropriate internal standards is the preferred approach.
지질 과산화
고도 불포화 지방산(PUFA)은 쉽게 산화되므로 지질 과산화 생성물은 산화 손상을 특성화하는 데 널리 사용됩니다56,57,58. 지질 과산화는 특정 ROS에 의해 시작될 수 있으며 임의의 비효소적(종종 연쇄적) 라디칼 과정으로 진행됩니다. 그러나 생물학적 역할을 하는 특정 신호 생성물을 생성하는 유리 PUFA 또는 PUFA 인지질의 과산화에 사용할 수 있는 효소 메커니즘(예: 리폭시게나제)도 있습니다. 따라서 지질 과산화를 측정할 때는 (1) 산화 손상의 예로서 지질 과산화 증가를 확인하거나 (2) 특정 세포 표적과 선택적으로 상호작용하여 신호로 작용하는 개별 산화 변형 지질 분자를 식별하는 데 초점을 맞출 수 있습니다.
PUFA에서 메틸렌기에 인접한 이중 결합의 존재는 메틸렌 C-H 결합을 약하게 만들고, 따라서 비스-알릴 수소는 H- 추상화에 더 취약합니다56,57,58. H- 추상화에 의해 생성된 탄소 중심 라디칼(L)은 이중 결합에 대한 탈위상화에 의해 안정화됩니다. 이후 O2와 반응하면 공액 디엔 시스템과 다양한 과산화물(LOOH)을 형성하는 과산화 라디칼(LOO)이 생성됩니다. LOO-는 추가로 반응하여 에폭시, 옥소 또는 순환 과산화물56,57,58을 포함한 고도로 산화된 이차 생성물을 생성할 수 있습니다. 따라서 지질 과산화에는 화학적 이질성과 다양한 안정성 및 극성을 보이는 여러 가지 최종 생성물이 존재하므로 단일 산화 생성물만을 측정하는 것은 지질 과산화의 전체 과정을 대표하지 못합니다.
'일반적인' 지질 과산화를 평가하기 위해 몇 가지 방법을 사용할 수 있습니다. 간단한 모델 시스템(예: 분리된 지단백질)에서는 자외선(UV) 흡광도로 디엔 접합을 측정할 수 있지만 이 방법은 지질 과산화로 인해 발생하지 않는 방해 자외선 흡수 분자의 존재로 인해 세포나 체액에서 사용하기에 적합하지 않습니다1. 세포에서 '지질 과산화'는 과산화에 민감한 운데카노산 모이티에 결합된 BODIPY의 형광 변화로 평가할 수 있습니다59. 이 분석법은 기술적으로 간단하지만, BODIPY가 과산화 라디칼과 반응하는 속도가 라디칼 제거 항산화제보다 느리기 때문에 항산화제에 의한 BODIPY 형광 억제가 항상 지질 과산화 억제 능력을 반영하는 것은 아니므로 신중하게 해석해야 합니다59. 지질 과산화에 대한 또 다른 형광 분석법은 4개의 공액 이중 결합을 가진 지방산인 시스-파리나르산(PnA)을 사용합니다. PnA의 산화는 공액 시스템을 방해하여 형광을 발산합니다. PnA는 여러 종류의 인지질에 통합될 수 있기 때문에 고성능 액체 크로마토그래피(HPLC) 분리를 통해 다양한 인지질의 산화에 대한 정보를 얻을 수 있습니다60. 그러나 PnA의 높은 산화 속도, 광표백에 대한 취약성, 다른 인지질로의 PnA의 다양한 대사적 통합으로 인해 PnA 기반 결과를 내인성 인지질 산화로 추정하는 것은 어렵습니다60.
지질 과산화는 α,β-불포화 하이드록시알케날61과 같은 최종 생성물의 측정으로 평가하는 경우가 많으며, 이상적으로는 MS 기반 기법을 통해 평가합니다. 특히 4-하이드록시노네날(HNE) 형성이 널리 사용되고 있습니다. HNE에 의해 형성된 단백질 부가물에 대한 항체는 널리 이용 가능하며 조직의 면역 염색에 자주 사용되지만, HNE가 단백질에서 어떤 아미노산 잔기와 결합하는지에 따라 다른 항체가 다른 에피토프를 감지할 수 있으므로 다른 답변을 제공한다는 것을 인식해야 합니다61,62,63,64.
지질 과산화의 부수적인 최종 생성물 중 하나는 말론다이알데히드(MDA)61이며, 이 또한 MS 기법으로 측정할 경우 유용한 바이오마커가 될 수 있습니다. 그러나 티오바르비투르산 반응성 물질(TBARS)을 활용하는 널리 사용되는 'MDA 분석법'은 TBA가 MDA 이외의 많은 생체 분자에서 염색질을 생성하기 때문에 비특이적입니다1,65. HPLC를 사용하여 '진짜' TBA-MDA 부가물과 가짜 염색체를 분리하면 특이도는 높아지지만 모든 문제가 제거되지는 않습니다1.
권고사항 11: 세포, 조직 또는 체액에 대한 간이 TBA 검사(TBARS) 또는 이를 기반으로 한 키트의 적용은 위양성 결과를 초래할 수 있는 낮은 특이도 때문에 산화성 지질 손상 평가에 사용되는 유일한 검사로 권장되지 않습니다. HPLC 기반 TBA 검사는 아티팩트 발생 가능성이 적습니다.
지질 산화 생성물의 검출은 산화된 지질 혼합물의 상세한 분석을 위한 LC-MS의 개발로 혁신적으로 발전했습니다66. 지질 과산화 연구의 핵심은 인공 과산화를 피하기 위한 시료의 수집과 보관이며, 추후 분석을 위해 시료는 즉시 액체 질소로 냉동 보관해야 합니다. 생체액은 보관 중 자가 산화를 방지하기 위해 화학 물질(예: 부틸화 히드록시톨루엔)을 첨가해야 할 수 있습니다1,67. 정량화에 사용되는 내부 표준물질은 용매 추출 전에 시료에 첨가해야 합니다. 이러한 LC-MS 기반 방법은 높은 감도, 적은 시료량 요구 사항 및 지질 과산화의 여러 최종 생성물을 검출할 수 있다는 장점이 있습니다. 따라서 LC-MS 프로토콜은 일반적인 지질 과산화를 평가하고 특정 신호 기능을 가진 제품을 포함한 개별 제품을 식별하기 위해 선택되는 방법입니다. 그러나 사용 가능한 표준의 제한으로 인해 특정 제품에 대한 정량 분석이 불가능한 경우도 있습니다. 이러한 연구에서는 방법론에 대한 세심한 주의가 필요합니다68.
MS 기반 접근법으로 정량화된 지질 산화 생성물 중 눈에 띄는 것은 F2-이소프로스탄(F2-IsoP)69입니다. 64개의 F2-IsoP 입체 이성질체는 아라키돈산의 자유 라디칼에 의한 비효소적 산화로부터 생성될 수 있으며, 시클로 옥시게나제 효소(COX-1/-2)에 의한 아라키돈산의 효소적 산화로부터 생성되는 것과 분리될 수 있습니다. F3- 및 F4- 이소프로스탄은 각각 에이코사펜타엔산(EPA)과 도코사헥사엔산(DHA)에서 발생하지만, F2- 이소프로스탄에 비해 그 특성이 잘 밝혀지지 않았습니다. 하나의 F2-IsoP 이성질체인 8-iso-PGF2α(15-F2t-IsoP 또는 iPF2α-III라고도 함)를 정량하는 ELISA 방법이 개발되었으며, 가스 크로마토그래피-MS 및 LC-텐덤 MS(LC-MS/MS) 방법69,70,71,72,73과 비교되었습니다. 이러한 모든 연구에서 시판되는 ELISA 키트와 MS 방법 간의 일치도가 낮았으며, 8-iso-PGF2α는 아라키돈산 과산화 과정에서 생성되는 64개의 서로 다른 F2-IsoP 이성질체 중 하나이며 8-iso-PGF2α와 관련 이성질체 간의 항체 교차 반응성이 까다롭습니다. 분석 전 시료 정화를 통해 ELISA72,73로 8-iso-PGF2α를 더 정확하게 측정할 수 있지만, F2-IsoP를 정량화하는 가장 정확한 방법은 LC-MS/MS를 사용하는 것이므로 이를 강력히 권장합니다.
권장 사항 12: F2-IsoP는 일반적으로 지질 과산화의 바이오마커로 인정되지만, 많은 최종 생성물 중 하나이며 다양한 유형의 수준이 실험 조건에 의해 영향을 받을 수 있다는 점을 인식해야 합니다69. ELISA를 사용한 정량화는 인공물에 영향을 받기 쉽지만, 시료 정화를 통해 8-iso-PGF2α를 ELISA로 측정할 수 있습니다73. 적절한 내부 표준을 사용하는 LC-MS/MS가 선호되는 접근 방식입니다
Protein damage
Amino acid residues in proteins are sensitive to oxidative modification, some forms of which provide useful biomarkers74,75. Detailed protocols for measurement of multiple products can be found in refs. 76,77. A common protein modification is the formation of ‘protein carbonyls’ due to oxidation of specific amino acid residues to carbonyl group-bearing products; carbonyls can also be formed by the reaction of aldehydes with nucleophilic sites on proteins or by glycation75,77. Many assays involve derivatization of the carbonyl group with 2,4-dinitrophenylhydrazine to form a dinitrophenylhydrazone (DNP). This product can be detected spectrophotometrically, although this approach can suffer from high background and low reproducibility; to circumvent this, DNP adducts can be separated by LC before measurement. Alternatively, carbonyls can be detected using an antibody against DNP products by ELISA or immunoblotting78. Changes in protein carbonyls can be measured in tissue homogenates treated with fluorescein-5-thiosemicarbazide (FTC) to generate fluorophore-labelled proteins that can be separated by gel electrophoresis79. Enrichment methods using biotin-tagged derivatization coupled with LC–MS detection have been developed80. Protein carbonyls, α-aminoadipic semialdehyde and glutamic semialdehyde have also been assayed individually by stable isotopic dilution analysis LC–MS/MS77. Of course, data from a single time point reflect the difference between the rates of formation and removal (for example, by repair or proteolysis) of these products.
Protein analysis using MS allows detection and identification of modifications with characteristic mass increases (for example, hydroxylation, nitration and chlorination)75,76. This has been particularly useful in studies of oxidative damage to brain proteins in patients with dementia by ‘redox proteomics’81. Peptide-level mapping after proteolytic cleavage allows detection of the nature of the modification, its location within the protein sequence and concomitant loss of the parent peptide, allowing relative quantification. Amino acid analysis after complete digestion allows determination of types and absolute concentrations of particular species (determined by the use of isotope-labelled standards) together with the parent species, allowing determination of a ‘mass balance’75,76,77,82. Care must be taken in sample handling to prevent artefactual oxidation of Cys or Met, and also during protein hydrolysis, because some products of oxidative protein damage are labile. LC–MS analyses have many advantages including high specificity, high sensitivity, the capacity to detect many different modifications and parent species concurrently, as well as the capacity to detect products that are diagnostic of the ROS involved, such as chlorination from HOCl83 and nitrated species arising from the action of myeloperoxidase in the presence of NO2− and/or by reactions of ONOO−/ONOOH49,84). These LC–MS approaches can be carried out on materials ranging from isolated proteins to tissue samples. Quantification relative to non-modified amino acids or peptides, and preferably against added heavy-isotope-labelled materials, is recommended to overcome potential artefacts arising from sample handling and preparation. However, do bear in mind that plasma and urinary levels of oxidized amino acids might have contributions from absorption of oxidized amino acids from proteins in food or from increased tissue proteolysis as a result of pathology. Neither of these have yet been studied in detail.
Cysteine is a major target for modification due to its ease of oxidation (particularly in its thiolate form, RS−) and its nucleophilicity, which results in ready adduct formation with electrophiles. Oxidation can be irreversible—for example, to a sulphinic or sulphonic acid, which can be useful biomarkers of oxidative protein damage4,85. Reversible oxidation of Cys residues in proteins is a prominent mechanism of redox signalling2,4. Reversible products include disulphides, sulphenic acids, S-nitrosothiol and persulphide species2,4,85,86,87. These modifications can be reversed by the GSH/glutaredoxin or thioredoxin systems87. A common approach in detection of the pool of reversibly modified Cys residues is to first block reduced thiols with a reactive reagent and then reduce and derivatize the previously oxidized residues with a tag that can be identified by LC–MS of tryptic digests88. These approaches can be extended to the use of modification-specific chemistry to tag only a particular oxidation product, such as an S-nitrosothiol, sulphenic acid or persulphide88. Until recently, major limitations to these approaches were low coverage of the total Cys pool and lack of quantitation of the modification at individual residues87,88,89. The latter is of particular importance when interpreting the biological importance of reversible modifications. Substantial improvements in quantification have been achieved by isobaric tagging, in which reduced Cys residues are first labelled with one tag, reversibly modified residues are reduced and then labelled with a chemically identical, but heavy-isotope-modified, tag that enables quantification of the proportion oxidized for each particular Cys. These methods have been extended to tags that incorporate moieties such as biotin, which enable enrichment of the labelled peptides, greatly enhancing Cys coverage. The most recent iteration of this approach, as exemplified by the OxiMOUSe study89, has superseded previous methods.
Methionine is also a major site of redox post-translational modifications. Oxidation to methionine sulphoxide can be reversed enzymatically by methionine sulphoxide reductase enzymes, potentially facilitating redox signalling by the installation/removal of a single oxygen atom17. Reagents have been developed for methionine bioconjugation that can identify and characterize redox-sensitive methionine sites in proteomes90.
단백질 손상
단백질의 아미노산 잔류물은 산화적 변형에 민감하며, 일부 형태는 유용한 바이오마커를 제공합니다74,75. 여러 제품의 측정을 위한 자세한 프로토콜은 참고 문헌에서 확인할 수 있습니다. 76,77. 일반적인 단백질 변형은 특정 아미노산 잔기가 카르보닐기를 갖는 생성물로 산화되어 '단백질 카르보닐'이 형성되는 것이며, 카르보닐은 알데히드와 단백질의 핵친화성 부위와의 반응 또는 당화75,77에 의해서도 형성될 수 있습니다. 많은 분석에서는 카르보닐기를 2,4-디니트로페닐히드라진으로 유도체화하여 디니트로페닐히드라존(DNP)을 형성합니다. 이 생성물은 분광광도법으로 검출할 수 있지만, 이 방법은 배경이 높고 재현성이 낮을 수 있으므로 이를 피하기 위해 측정 전에 DNP 부가물을 LC로 분리할 수 있습니다. 또는 DNP 생성물에 대한 항체를 사용하여 ELISA 또는 면역 블 롯팅78으로 카르보닐을 검출할 수 있습니다. 단백질 카르보닐의 변화는 플루오레세인-5-티오세미카바자이드(FTC)로 처리한 조직 동질체에서 측정하여 겔 전기영동으로 분리할 수 있는 형광 표지 단백질을 생성할 수 있습니다79. 비오틴 태그 유도체화와 LC-MS 검출을 결합한 농축 방법이 개발되었습니다80. 단백질 카르보닐, α-아미노아디픽 세미알데히드 및 글루탐 세미알데히드도 안정 동위원소 희석 분석 LC-MS/MS77에 의해 개별적으로 분석되었습니다. 물론 단일 시점의 데이터는 이러한 제품의 형성 속도와 제거 속도(예: 복구 또는 단백질 분해에 의한)의 차이를 반영합니다.
MS를 사용한 단백질 분석은 특징적인 질량 증가(예: 하이드 록실화, 질화 및 염소화)75,76와 같은 변형을 감지하고 식별할 수 있습니다. 이는 특히 치매 환자의 뇌 단백질에 대한 산화적 손상을 '산화 환원 단백질체학'으로 연구하는 데유용합니다81. 단백질 분해 절단 후 펩타이드 수준 매핑을 통해 변형의 특성, 단백질 서열 내 위치 및 모 펩타이드의 수반되는 손실을 감지하여 상대적인 정량화를 가능하게 합니다. 완전 분해 후 아미노산 분석을 통해 모종과 함께 특정 종의 유형 및 절대 농도(동위원소 표지 표준을 사용하여 결정)를 측정하여 '질량 균형'75,76,77,82을 결정할 수 있습니다. 산화성 단백질 손상의 일부 생성물은 불안정하기 때문에 Cys 또는 Met의 인공 산화를 방지하고 단백질 가수분해 중에도 시료 취급에 주의를 기울여야 합니다. LC-MS 분석은 높은 특이성, 높은 감도, 다양한 변형과 모종을 동시에 검출할 수 있는 능력, 그리고 NO2- 및/또는 ONOO-/ONOOH의 반응에 의해 발생하는 HOCl83 및 미엘로퍼옥시다아제의 작용으로 발생하는 질산화된 종과 같은 관련 ROS를 진단하는 제품을 검출할 수 있는 능력 등 많은 장점을 가지고 있습니다49,84). 이러한 LC-MS 접근법은 분리된 단백질부터 조직 샘플까지 다양한 물질에 대해 수행할 수 있습니다. 시료 취급 및 준비로 인해 발생할 수 있는 잠재적 인공물을 극복하기 위해 변형되지 않은 아미노산 또는 펩타이드에 대한 정량화, 가급적 추가된 중동위원소 표지 물질에 대한 정량화를 권장합니다. 그러나 혈장 및 소변의 산화 아미노산 수치는 음식의 단백질에서 산화된 아미노산이 흡수되거나 병리로 인한 조직 단백질 분해 증가로 인해 영향을 받을 수 있다는 점을 염두에 두어야 합니다. 이 중 어느 것도 아직 자세히 연구되지 않았습니다.
시스테인은 산화가 용이하고(특히 티올레이트 형태인 RS-) 핵친화성이 있어 친전기로 쉽게 부가물을 형성하기 때문에 변형의 주요 표적입니다. 예를 들어, 산화 단백질 손상의 유용한 바이오마커가 될 수 있는 황산 또는 설폰산으로의 산화는 비가역적일 수 있습니다4,85. 단백질 내 Cys 잔기의 가역적 산화는 산화 환원 신호의 중요한 메커니즘입니다2,4. 가역적 생성물에는 이황화물, 설펜산, S-니트로소티올 및 퍼술파이드 종2,4,85,86,87이 포함됩니다. 이러한 변형은 GSH/글루타레독신 또는 티오레독신 시스템에 의해 되돌릴 수 있습니다87. 가역적으로 변형된 Cys 잔기의 풀을 검출하는 일반적인 접근법은 먼저 반응성 시약으로 환원된 티올을 차단한 다음 트립틱 다이제스트의 LC-MS로 식별할 수 있는 태그를 사용하여 이전에 산화된 잔기를 환원 및 유도체화시키는 것입니다88. 이러한 접근법은 변형 특이적 화학을 사용하여 S-니트로소티올, 설펜산 또는 퍼술파이드와 같은 특정 산화 생성물에만 태그를 지정하는 것으로 확장할 수 있습니다88. 최근까지 이러한 접근법의 주요 한계는 전체 Cys 풀의 낮은 커버리지와 개별 잔기에서의 변형 정량 부족이었습니다87,88,89. 후자는 가역적 변형의 생물학적 중요성을 해석할 때 특히 중요합니다. 환원된 Cys 잔기를 먼저 하나의 태그로 라벨링하고 가역적으로 변형된 잔기를 환원시킨 다음 화학적으로 동일하지만 중동위원소로 변형된 태그로 라벨링하여 각 특정 Cys의 산화 비율을 정량화할 수 있는 등압 태깅을 통해 정량화의 상당한 개선이 이루어졌습니다. 이러한 방법은 표지된 펩타이드를 농축할 수 있는 비오틴과 같은 모오티를 통합하는 태그로 확장되어 Cys 커버리지를 크게 향상시켰습니다. 이 접근법의 가장 최근 반복은 OxiMOUSe 연구89에서 예시된 바와 같이 이전 방법을 대체했습니다.
메티오닌은 또한 번역 후 산화 환원 변형의 주요 부위입니다. 메티오닌 설폭사이드로의 산화는 메티오닌 설폭사이드 환원 효소에 의해 효소적으로 역전될 수 있으며, 단일 산소 원자의 설치/제거를 통해 산화 환원 신호 전달을 촉진할 가능성이 있습니다17. 프로테옴에서 산화 환원에 민감한 메티오닌 부위를 식별하고 특성화할 수 있는 메티오닌 생체 접합용 시약이 개발되었습니다90.
Recommendation 13: ELISA, FTC and immunoblotting are useful tools in the detection of protein carbonyls as a biomarker of general oxidative protein damage, although it must be realized that not all protein oxidation products contain carbonyls. LC–MS approaches, using carefully prepared samples, are the best available techniques for assessment of protein oxidation due to the sensitivity, selectivity and quantitation available with these methods. The use of orthogonal approaches, such as specific and validated antibodies (see below) against individual oxidation products, is also encouraged.
권장 사항 13: 모든 단백질 산화 생성물에 카르보닐이 포함되어 있는 것은 아니지만, ELISA, FTC 및 면역 블 롯팅은 일반적인 산화성 단백질 손상의 바이오마커로서 단백질 카르보닐을 검출하는 데 유용한 도구입니다. 신중하게 준비된 시료를 사용하는 LC-MS 접근법은 감도, 선택성 및 정량성이 우수하기 때문에 단백질 산화를 평가하는 데 가장 적합한 기술입니다. 개별 산화 생성물에 대한 특이적이고 검증된 항체(아래 참조)와 같은 직교 접근법을 사용하는 것도 권장됩니다.
Nucleic acids
Oxidative modifications of DNA and RNA are often used as biomarkers of oxidative damage1,13,91. One method used to assess ‘general’ oxidative damage to DNA in cells is the comet assay, which detects DNA strand breaks. Such breaks can arise by several mechanisms, not necessarily via oxidative damage, but the use of repair enzymes that ‘nick’ DNA at the sites of oxidation increases the specificity for oxidative DNA damage. The simplest measurement is the length of the DNA ‘ghost’ following electrophoresis of cells embedded on a gel on a microscope slide92.
Oxidative damage to DNA usually focuses on oxidation of guanine to 8-oxo-7,8-dihydro-2′-deoxyguanosine (8OHdG, or 8-oxodG). Data on modifications at other bases are limited, although these are likely to be biologically important1,13. These measurements require the isolation of the DNA and its digestion to release modified bases, and there can be spurious oxidation during sample handling and analysis. Multi-laboratory initiatives93 have established protocols to avoid this and have determined ‘normal’ levels of 8OHdG. The amount of 8OHdG (or any other product of oxidative DNA damage) measured in DNA is the balance between the rate of oxidation and that of repair. The best methodology is ultra-performance LC–MS/MS (UPLC–MS/MS)94. Caution should be exercised in using ELISA methods, which lack sensitivity and specificity and can give variable results between batches, and there is sometimes cross-reaction between 8-hydroxyguanosine (8OHG) and 8OHdG. However, immunohistochemistry can be useful in identification of cells that have higher amounts of 8-OHdG in vivo, if applied appropriately95.
Oxidized nucleosides from both DNA and RNA can be detected in various body fluids. Originally they were believed to arise from DNA repair, particularly nucleotide excision repair. However, they also arise from oxidation of the DNA and RNA nucleotide precursor pools ‘sanitized’ by removal of oxidized products94. The relative contributions of DNA repair and nucleotide pool sanitization to the levels of oxidized nucleosides detected in body fluids are currently unclear. Urine collected over 24 h will represent the number of guanines in DNA/RNA and/or the respective nucleotide precursor pools oxidized during that period96. Urine sampling represents formation within the entire body, and is best suited to situations where all tissues are assumed to be affected, but it could be inadequate in the detection of changes that occur only in certain organs. Measurement in specific tissues will be a snapshot of the balance between generation and repair, and may not represent processes in other organs.
Recommendation 14: when measuring oxidative modifications of nucleic acids from extracted cells or tissue samples, great care must be taken to avoid spurious oxidation in the preparative and analytical steps. Methods such as the comet assay (using DNA repair enzymes) on isolated cells and UPLC–MS/MS for 8OHdG and 8OHG determination in body fluids or nucleic acids extracted from tissues are presently the best available. ELISA-based methods, especially in kit form, are usually insufficiently validated and their use is not recommended.
핵산
DNA와 RNA의 산화적 변형은 종종 산화적 손상의 바이오마커로 사용됩니다1,13,91. 세포의 DNA에 대한 '일반적인' 산화 손상을 평가하는 데 사용되는 한 가지 방법은 DNA 가닥 단절을 감지하는 혜성 분석법입니다. 이러한 단절은 반드시 산화적 손상이 아닌 여러 메커니즘에 의해 발생할 수 있지만, 산화 부위에서 DNA를 '닉'하는 복구 효소를 사용하면 산화적 DNA 손상에 대한 특이성이 증가합니다. 가장 간단한 측정 방법은 현미경 슬라이드의 젤에 묻힌 세포를 전기영동한 후 DNA '고스트'의 길이를 측정하는 것입니다92.
DNA의 산화적 손상은 일반적으로 구아닌이 8-옥소-7,8-디하이드로-2′-데옥시구아노신(8OHdG 또는 8-옥소드G)으로 산화되는 데 중점을 둡니다. 다른 염기의 변형에 대한 데이터는 생물학적으로 중요할 가능성이 있지만 제한적입니다1,13. 이러한 측정을 위해서는 DNA를 분리하고 변형된 염기를 방출하기 위해 분해해야 하며, 샘플 취급 및 분석 중에 가짜 산화가 발생할 수 있습니다. 여러 실험실 이니셔티브93에서는 이를 방지하기 위한 프로토콜을 수립하고 8OHdG의 '정상' 수준을 결정했습니다. DNA에서 측정된 8OHdG(또는 기타 산화성 DNA 손상의 산물)의 양은 산화 속도와 복구 속도 사이의 균형입니다. 가장 좋은 방법론은 초고성능 LC-MS/MS(UPLC-MS/MS)94입니다. 민감도와 특이도가 부족하고 배치 간에 다양한 결과를 제공할 수 있으며 때때로 8-하이드록시구아노신(8OHG)과 8OHdG 사이에 교차 반응이 있는 ELISA 방법을 사용할 때는 주의를 기울여야 합니다. 그러나 면역조직화학을 적절히 적용하면 생체 내에서 더 많은 양의 8-OHdG를 가진 세포를 식별하는 데 유용할 수 있습니다95.
DNA와 RNA 모두에서 산화된 뉴클레오시드는 다양한 체액에서 검출될 수 있습니다. 원래 뉴클레오시드는 DNA 복구, 특히 뉴클레오티드 절제 복구에서 발생하는 것으로 여겨졌습니다. 그러나 이들은 또한 산화된 산물을 제거하여 '살균'된 DNA 및 RNA 뉴클레오티드 전구체 풀의 산화로 인해 발생합니다94. 체액에서 검출되는 산화된 뉴클레오시드의 수준에 대한 DNA 복구와 뉴클레오티드 풀 살균의 상대적인 기여도는 현재 불분명합니다. 24시간 동안 채취한 소변은 해당 기간 동안 산화된 DNA/RNA 및/또는 각 뉴클레오타이드 전구체 풀의 구아닌 수를 나타냅니다96. 소변 샘플링은 전신의 형성을 나타내며 모든 조직이 영향을 받는다고 가정하는 상황에 가장 적합하지만 특정 장기에서만 발생하는 변화를 감지하는 데는 부적절할 수 있습니다. 특정 조직에서의 측정은 생성과 복구 사이의 균형에 대한 스냅샷이며 다른 장기의 과정을 나타내지 않을 수 있습니다.
권장 사항 14: 추출한 세포 또는 조직 샘플에서 핵산의 산화적 변형을 측정할 때는 준비 및 분석 단계에서 가짜 산화를 피하기 위해 세심한 주의를 기울여야 합니다. 현재로서는 분리된 세포에 대한 혜성 분석(DNA 복구 효소 사용), 체액 또는 조직에서 추출한 핵산에서 8OHdG 및 8OHG 측정을 위한 UPLC-MS/MS와 같은 방법이 가장 많이 사용되고 있습니다. 특히 키트 형태의 ELISA 기반 방법은 일반적으로 검증이 충분하지 않으므로 사용을 권장하지 않습니다.
Some general comments on antibodies
As discussed above, antibodies have been widely used to detect oxidation products (and also adducts) formed on proteins (for example, carbonyls and 3-nitro- and 3-chlorotyrosine), DNA (for example, 8-oxodG) and lipids (F2-Isoprostanes). They have been used, for example, in ELISA, immunohistochemistry and immune precipitation formats, but often suffer from background reactivity, cross-reactivity and lack of specificity. To address this, the epitope used to generate the antibody should be documented (for example, as for HNE)62,63,64 and controls to eliminate background should be included. Blocking by authentic samples of the epitope is recommended to determine selectivity. Relative quantification is possible but absolute quantification can be difficult—for example, due to poor epitope accessibility (for example, in proteins the oxidation product may be buried). In addition, antibodies are typically generated against unstructured, chemically modified peptides and the epitope(s) recognized may not always have been determined.
항체에 대한 몇 가지 일반적인 의견
위에서 설명한 대로 항체는 단백질(예: 카르보닐 및 3-니트로 및 3-클로로티로신), DNA(예: 8-옥소드G) 및 지질(F2-이소프로스탄)에 형성된 산화 생성물(및 부가물)을 검출하는 데 널리 사용되어 왔습니다. 예를 들어 ELISA, 면역조직화학 및 면역 침전 형식으로 사용되어 왔지만 배경 반응성, 교차 반응성 및 특이성 부족으로 인해 종종 어려움을 겪습니다. 이를 해결하려면 항체를 생성하는 데 사용된 에피토프를 문서화해야 하며(예: HNE)62,63,64 배경을 제거하기 위한 대조군을 포함해야 합니다. 선택성을 결정하기 위해 에피토프의 실제 샘플로 차단하는 것이 좋습니다. 상대 정량화는 가능하지만 절대 정량화는 어려울 수 있습니다(예: 단백질의 경우 산화 산물이 묻혀 있을 수 있음) - 예를 들어 에피토프 접근성이 좋지 않을 수 있습니다. 또한 항체는 일반적으로 구조화되지 않은 화학적으로 변형된 펩타이드에 대해 생성되며 인식되는 에피토프가 항상 결정되지 않을 수 있습니다.
Recommendation 15: well-validated antibodies against specific products are useful detection tools when used with appropriate care and controls, including those for non-specific interactions. Competition data with authentic epitopes should be included whenever possible.
Measurement of ROS and oxidative damage in vivo
Measurement of ROS in vivo is a challenge. EPR methods have been developed but are not yet widely used. Bioluminescent approaches to ROS detection include peroxy-caged luciferin-1 which, upon oxidation, forms luciferin in situ that is oxidized in luciferase-transfected systems to generate bioluminescence97. As noted earlier, genetically encoded redox biosensors have been used in animal studies. With the development of improved sensitivity and detection modalities, positron emission tomography is now being used to image ROS in vivo98 but is still in its infancy. In mitochondria of cells and tissues, changes in H2O2 can be assessed using the mitochondria-targeted boronate MitoB, which accumulates in these organelles and is converted by H2O2 into MitoP. The ratio of MitoP to MitoB can then be determined by MS99.
Measurement of ROS and oxidative damage in clinical trials
Because oxidative damage plays a central role in many human pathologies, there is considerable interest in developing therapeutic interventions to decrease this damage1,2,3. A corollary is that in clinical trials we should be able to demonstrate how these interventions affect oxidative damage. For example, many double-blinded randomized clinical trials have been conducted using ‘antioxidants’ such as beta-carotene, vitamin C and vitamin E. These generally failed to influence disease activity. Unfortunately, in most cases the effect of the intervention on oxidative damage was not measured, making it uncertain whether the putative therapy was actually effective at decreasing oxidative damage: if it was not, lack of effect is predictable1,55.
To address this, it is essential to assess the impact of these interventions on levels of oxidative damage in the patients in clinical trials. Currently, methods are limited to measuring end points of oxidative damage in either biopsies (for example, skin or muscle) or clinically accessible body fluids such as plasma, saliva, sputum or urine, and sometimes cerebrospinal fluid. These biomarkers have included those for oxidation of nucleic acids such as 8OHG and 8OHdG100 and F2-isoprostanes as a biomarker of lipid peroxidation69,101. To date, limited use has been made of biomarkers of protein oxidation in clinical trials. However, there is evidence for strong associations of alterations in protein thiol/disulphide ratios, and increased protein carbonyls and other modifications with pathologies75,76,77.
More generally, clinical trials should include internationally validated biomarkers: the biomarker should ideally have undergone interlaboratory comparison. Many biomarkers rely on concentration measurement in body fluids such as plasma, but these reflect only the balance between formation and elimination rates and therefore cannot readily be interpreted as ‘oxidative stress’. However, models have been developed to estimate the 24 h production of certain biomarkers100. Ideally a panel of biomarkers should be used54,55 since end products of oxidative damage to lipids, proteins and nucleic acids do not necessarily correlate with each other, nor would we expect them to since they are different molecular targets of different ROS.
Recommendation 16: if intervening with antioxidants, first use biomarkers in preliminary dose-ranging studies to determine whether the intervention does indeed decrease oxidative damage to the relevant biomolecules. They should include well-defined biomarkers analysed with a validated methodology and/or orthogonal approaches. We do not recommend in clinical (or other!) studies the use of the d-ROMS assay (for reasons explained in ref. 1), TBARS, determinations of total antioxidant activity1,102 or kit-based methods where the methodology behind the kit is not clear and/or has not been validated.
Concluding remarks
The goal of this consensus statement is to generate a useful resource for researchers from diverse fields who find themselves needing to measure ROS and to assess oxidative events to investigate their biological importance. We have discussed the limitations of many of the procedures currently used and suggested the best currently available approaches. Inevitably, new techniques will be developed and applied in the future, but the principles of our cautious philosophy, illustrated by our 16 recommendations (summarized in Fig. 1), will remain valid.
Fig. 1: Summary of recommendations for measurement of ROS.
Here we have summarized and abbreviated the recommendations for best practices developed in this manuscript.
References